the Creative Commons Attribution 4.0 License.
the Creative Commons Attribution 4.0 License.
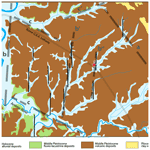
A morphotectonic approach to the study of earthquakes in Rome
Fabrizio Marra
Alberto Frepoli
Dario Gioia
Marcello Schiattarella
Andrea Tertulliani
Monica Bini
Gaetano De Luca
Marco Luppichini
Rome has the world's longest historical record of felt earthquakes, with more than 100 events during the last 2600 years. However, no destructive earthquake has been reported in the sources, and all of the greatest damage suffered in the past has been attributed to far-field events. While this fact suggests that a moderate seismotectonic regime characterizes the Roman area, no study has provided a comprehensive explanation for the lack of strong earthquakes in the region. Through the analysis of the focal mechanism and the morphostructural setting of the epicentral area of a “typical” moderate earthquake (Ml= 3.3) that recently occurred in the northern urban area of Rome, we demonstrate that this event reactivated a buried segment of an ancient fault generated under both a different and a stronger tectonic regime than that which is presently active. We also show that the evident structural control over the drainage network in this area reflects an extreme degree of fragmentation of a set of buried faults generated under two competing stress fields throughout the Pleistocene. Small faults and a present-day weaker tectonic regime with respect to that acting during the Pleistocene might explain the lack of strong seismicity in the long historical record, suggesting that a large earthquake is not likely to occur.
- Article
(19180 KB) - Full-text XML
- BibTeX
- EndNote
On 11 May 2020, a moderate (Ml= 3.3, Io = IV MCS) yet broadly felt earthquake awoke most of Rome's inhabitants at 05:03 (local time) (for details see https://e.hsit.it/24397691/index.html, last access: 23 July 2022). While producing no damage, the shaking alarmed many citizens, who searched for information and reassurance through the dedicated informative sources such as the INGV (Italian National Institute of Geophysics and Volcanology) website. Others, instead, preferred to trust several popular beliefs which state that “Rome couldn't be struck by a Big One” (i.e., a destructive earthquake with M > 7.0), such as the mitigating effect of the catacomb voids (trivial simplification from the Aristotelian theories) or the protection granted by the Pope's presence. It is very likely that only a few people based their reactions upon knowledge learned of the actual seismotectonic features of Rome's area. Indeed, even if a series of specialized studies had been published in the last 20 years, a dedicated paper investigating the reasons why Rome would not be affected by large earthquakes is still missing in the scientific literature. Filling this gap is the aim of the present paper in which we present a seismic study of the 11 May 2020 earthquake, coupled with a statistical analysis of streambed directions in the epicentral area. We identify the geometry of the seismogenic structure responsible for this M= 3.3 event, and we frame it within the overall geo-morphostructural setting of Rome's area, providing insights on the seismotectonic features of this region.
Our knowledge of the earthquakes that affected the Roman area can be resumed from the seismic catalogues' records (Guidoboni et al., 2018; Rovida et al., 2020) and from the literature (e.g., Tertulliani and Riguzzi, 1995; Molin and Rossi, 2004; Galli and Molin, 2014; Tertulliani et al., 2020) as follows.
-
Very few events caused significant damage in the city (1349, 1703, 1915), according to the studies mentioned above; all these large earthquakes occurred in the Apennine mountain range.
-
Some other seismogenic areas surrounding Rome (e.g., the Colli Albani volcanic district) generated events that caused moderate damage.
-
The Province of Rome (hereafter G.R.A., the present metropolitan area of Rome) is periodically affected by low- to moderate-magnitude local earthquakes which are not supposed to cause significant damage.
-
For uncertain events, catalogue records quote several earthquakes that resulted in some damage in Rome (see Table 1). Most of such events, occurring during the Roman Age and Early Middle Ages, are poorly documented and therefore not localizable.
A summary of the historical and instrumental seismicity of the G.R.A. is shown in Fig. 1. Evidently, the completeness of our knowledge of seismicity decreases going back in time. In the period of ancient Rome, as well in the Early Middle Ages, strong earthquakes would seem to hit Rome, sometimes causing damage, whose origin is still unknown. The difficulty to understand if such earthquakes were generated by local or far-field sources depends on the documentary accounts: the earthquake was considered a prodigy and as such was interpreted as a divine foretelling. Information on effects, damage or victims was often neglected and very rarely documented. For these reasons we are not able to distinguish with reliability if such ancient events were originated, for example, in the Apennine region or near Rome (in italic in Table 1). In Table 1 the earthquakes that hit Rome with a local intensity greater than 6 are listed.
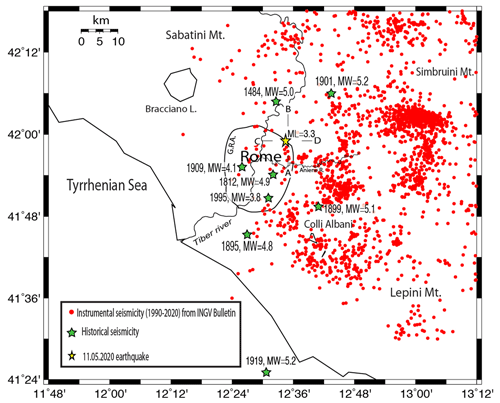
Figure 1Map showing the seismicity of Rome's area and mainshock location (yellow star) of the 11 May 2020 earthquake. A–B and C–D are the cross-sections in Fig. 4b. G.R.A. is the beltway around Rome.
Table 1List of earthquakes that caused documented damage in the present G.R.A. The oldest events (italic in table) are not constrainable. (Data from Guidoboni et al., 2018; Rovida et al., 2020; Tertulliani et al., 2020.)
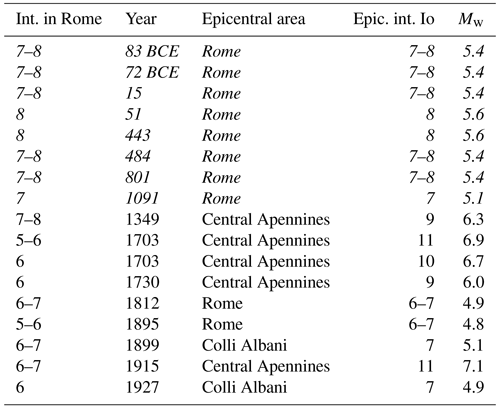
It is interesting to note, from the seismic hazard point of view, that the epicenter of several more constrainable historical events that occurred in the Roman countryside are nowadays included in the densely urbanized G.R.A. territory.
Within this limited territory we can in any case discriminate some different clusters of seismicity, in particular SE and NE of the city center. Of the first cluster there are the 1812, 1895 and 1995 earthquakes, while the 1901 and 2020 events are located NE of the city (Fig. 1). Very likely this seismicity feature is due to the activity of different seismotectonic structures.
In approaching the geodynamics of this region the contribution of three main mechanisms of deformation should be considered, as proposed in Faccenna et al. (1996):
- i.
the NE–SW shortening (arrow no. 1 in Fig. 2b) induced by the convergence of Africa and Europe (Tapponnier, 1977);
- ii.
the sinking of the Ionian slab (arrow no. 2 in Fig. 2b), producing the eastward migration (arrow no. 3) of the Apennine arc and consequent back-arc extension (arrow no. 4) in the Tyrrhenian region (Malinverno and Ryan, 1986; Patacca and Scandone, 1989);
- iii.
the gravitational spreading of the overthickened crust (arrow no. 5 in Fig. 2b) in the Apennine crustal wedge (Reutter et al., 1980; Horvath and Berckhemer, 1982).
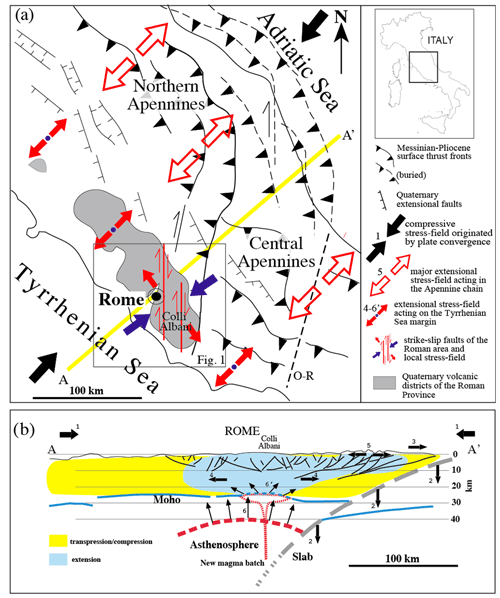
Figure 2Structural scheme of central Italy: map (a) and cross-section (b) showing the competing tectonic force fields and the main faults associated with them that acted in the Middle–Upper Pleistocene. See text for comments and explanations.
All these mechanisms are to be considered presently active in the northern Apennine arc on the basis of seismic and stress-field indications (Selvaggi and Amato, 1992; Amato et al., 1993; Frepoli and Amato, 1997; Mariucci et al., 1999; Lucente and Speranza, 2001; Montone and Mariucci, 2016). Moreover, crustal thinning induced by extension was coupled with asthenospheric bulging (arrows no. 6 in Fig. 2b), leading to the back-arc volcanism on the Tyrrhenian margin (Serri, 1997, and references therein). Such phenomena, and related magma underplating, enhanced the extensional processes (arrow no. 6" in Fig. 2b) in a feedback mechanism in this region. In this regard, it is fundamental to notice that the Roman area and the Colli Albani are at the southeastern margin of the Latium Magmatic Province (Serri et al., 1993), and that very scant volcanic activity occurred in the area between Rome and the Ortona-Roccamonfina line (O-R in Fig. 2a), which is considered (Patacca and Scandone, 1989) a major geodynamic boundary separating the central and southern Apennines (Fig. 2a). According to Marra (1999, 2001), the Sabina shear zone (Alfonsi et al., 1991) represents the northern boundary of this crustal disengagement zone. Based on its proximity to the Sabina shear zone, and in agreement with the numerous field evidence of fault kinematics (Faccenna et al., 1994a, b; Marra, 2001; Marra et al., 2004b) and the peculiar eruptive behavior of the Colli Albani volcanic district (Marra et al., 2009), Frepoli et al. (2010) proposed that the transpressional stress regime has been the prevailing one in this region during Quaternary times and that it is temporarily superimposed by the extensional regime during periods of incoming volcanic activity and/or increased extensional activity (depending on which is to be considered cause and which effect) on the Tyrrhenian margin (Fig. 2b).
The morphostructural setting of the Roman area originates in the deformation of the geological substrate by combined faulting processes and erosion of rivers and streams (Del Monte et al., 2016). Although partially obliterated by millennia of anthropic interventions, it presents some evident and peculiar traits, whose analysis allows us to understand the features of the tectonic forces (and related stress fields) that acted from the geological past through the present time (Marra, 2001) (Fig. 2). Such an analysis also allows us to interpret the origin of the earthquakes that nowadays affect this area.
If we could see what the topography was like before the foundation of the city, the area of Rome would appear as a large flat sector, deeply engraved and dissected by the valleys of the tributary streams of the Tiber and Aniene rivers, as well as by the wider ones of the two main watercourses. While these features are less visible in the historical center of Rome, they are still well recognizable through a digital elevation model (DEM) of its surrounding territory, as highlighted in Fig. 3.
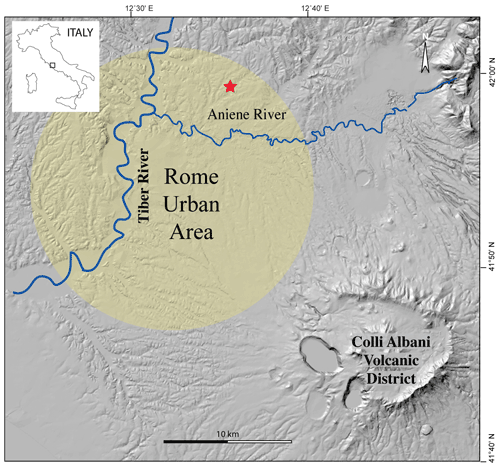
Figure 3Digital elevation model (DEM) of the Roman area (TINITALY by Istituto Nazionale di Geofisica e Vulcanologia (INGV), published with a CC BY 4.0 license; available at: https://doi.org/10.13127/TINITALY/1.0), showing the strongly marked characteristics of the river and stream incisions that form the hydrographic network of tributary streams which flow towards (into) the main Tiber and the Aniene rivers. Location of the 11 May 2020 earthquake is also shown (red star).
Most of the tabular surface highlighted by the shaded area in Fig. 3 is a “pyroclastic plateau” created by the emplacement of thick coulters of volcanic deposits erupted from the Colli Albani and Monti Sabatini districts. These are represented by pyroclastic flows, originated by the collapse of the sustained eruptive column, and air-fall products such as windblown pumice, scoria and lapilli. The deposition of these volcanic products, starting from around 600 000 years ago (Marra et al., 2014; Gaeta et al., 2016), leveled the ground creating a thick, layered blanket of sediments which was soon after etched by the erosive action of the watercourses. The latter, however, did not settle at random but progressively shifted in correspondence with embryonic fractures and fault lines created by active tectonic deformation. The same fracturing and faulting associated with the extensional tectonic regime which shaped the Tyrrhenian Sea margin of central Italy during the Pleistocene allowed the magma residing in the mantle to rise to the surface (e.g., Locardi et al., 1977; Acocella and Funiciello, 2006), originating the volcanoes of the so-called “Roman Province” (Peccerillo, 2017) (Fig. 2). An intense seismotectonic regime must have been associated with these large extensional faults, likely producing strong earthquakes throughout this region.
From the end of the Middle Pleistocene (125 000 years ago), the tectonic activity began to decrease in intensity, paralleling the decrease in volcanic activity (Marra et al., 2004a). Hence the seismogenic potential of the faults associated with this tectonic regime must also have decreased significantly. This is one of the reasons why Rome is today a low-seismicity area. Moderate earthquakes (M≤5.0) (Tertulliani and Riguzzi, 1995; Basili et al., 1996) are almost exclusively concentrated in the volcanic area of Colli Albani (Amato and Chiarabba, 1995), which is in a quiescent status (Trasatti et al., 2018). The moderate seismicity of the Roman area reflects an active stress field of the same nature, but weaker, than the extensive tectonic regime that characterized the Tyrrhenian Sea margin of central Italy for the entire Pleistocene, as revealed by the study of the focal mechanisms of these earthquakes and borehole breakouts (Montone et al., 1995; Montone and Mariucci, 2016). Such a weaker tectonic regime, therefore, reactivates all the faults present in this region with small movements that are compatible with their orientation with respect to the vectors of the stress field (Frepoli et al., 2010). The seismic events associated with this regime do not generate ground ruptures, as happens for strong, very destructive earthquakes, because the small displacements that occur on the fault planes at depth do not propagate to the surface. However, these movements repeated over time generate a slow and progressive deformation of the soil, conditioning the flow direction of surface waters and exerting a “structural control” on the stream axes and alluvial valleys (Marra, 2001). It follows that the hydrographic network has assumed over time a geometry reflecting that of the faults occurring in the geological substrate.
5.1 Seismic analysis
The small seismic sequence occurring on 11 May 2020 in the northeastern area of Rome was recorded by the Italian National Seismic Network (RSN) of the Istituto Nazionale di Geofisica e Vulcanologia (INGV) and by the regional seismic network of Lazio and Abruzzo (RSA) (De Luca et al., 2009; Frepoli et al., 2017) (Fig. 4). Both national and regional Italian seismic networks have been significantly extended in the last two decades through installation of three new components – mostly broadband stations. In addition we integrated the dataset of this sequence with the data of the Italian Strong Motion Network (RAN) operated by the National Civil Protection Department and with the IESN (Italian Experimental Seismic Network) of Central Italy, an amateur seismic network equipped with very good digitizers and sensors. This dense monitoring improved in the last decade the detection and location of the seismicity in central Italy.
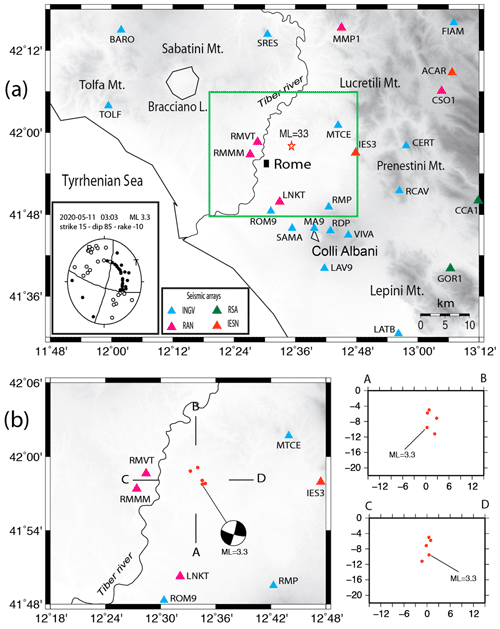
Figure 4(a) Distribution of the seismic stations of the Italian National Seismic Network (RSN) of the Istituto Nazionale di Geofisica e Vulcanologia (INGV) and of the regional seismic network of Lazio and Abruzzo (RSA) used to locate the epicenter of the 11 May 2020 event (red star). (b) Map and vertical distribution of the mainshock and two aftershocks.
To accurately relocate the seismicity, we used the Hypoellipse code (Lahr, 1989) and a reliable 1D Vp velocity model computed by the application of a genetic algorithm (Holland, 1975; Sambridge and Gallagher, 1993). A constant value of 1.84 determined with the Wadati method (Chatelain, 1978) was used.
5.2 Geomorphology
5.2.1 Previous studies
A quantitative analysis of drainage trends in the southeastern area of Rome bounded by the Tiber and Aniene rivers and by the Colli Albani volcanic district was carried out by Marra (2001). A simple technique based on statistical analysis of rectified directions of streambeds was applied (e.g., Ciccacci et al., 1987; Caputo et al., 1993; Macka, 2003). Stream channel directions for the total area and for different sectors were weighted according to three groups of length, independent of hydrographic order, and plotted on rose diagrams.
While it is possible that rectifying drainage patterns can introduce directionality that is unrelated to structural control, it still does indicate preferential directions of river flow. In the case that these preferential directions of river flow were statistically significant and different from those expected from non-structural controls (e.g., topographic and geographic trends), they were interpreted to be diagnostic of the structural setting. Anthropic intervention is also a possible cause of rectification of water channels; however, the linearity of the alluvial valleys forming in the hydrographic network allows us to compare and support the directionality of the streambeds. Indeed, deep incisions and a “canyon-like” morphology characterize the alluvial plains forming the hydrographic network (see Fig. 3) due to the occurrence of ca. 50 m tectonic uplift in the last 250 kyr (Marra et al., 2016).
Results of the analysis conducted by Marra (2001) are shown in Fig. 5b showing that the NW–SE direction is the dominant one in the total area analysis (large diagram in the upper-left corner), as opposed to an expected radial drainage trend descending from the Colli Albani caldera rim and affecting an heterogeneous geologic substrate. The maximum concentration of fluvial channel directions oriented 145∘N matches the strike of extension-induced faults and fractures and agrees with the present-day stress field determined from focal mechanisms and breakout data in this region (Montone et al., 1995; Montone and Mariucci, 2016). Moreover, there are significantly different concentrations in discrete sectors delimited by the yellow lines. In particular, there are two narrow bands (zones 2 and 4) where the N–S direction of the streambeds prevails and peculiar “domains” (zones 1A, 5A) where the WNW–ESE one is prevailing. The validation of the “tectonic” hypothesis was performed through comparison with geometry and kinematics of fault and fractures surveyed in the area, allowing us to interpret the pattern highlighted as the result of a complex structural control in this area exerted by two competing stress fields alternating with each other throughout Pleistocene times (Marra, 1999, 2001; Frepoli et al., 2010).
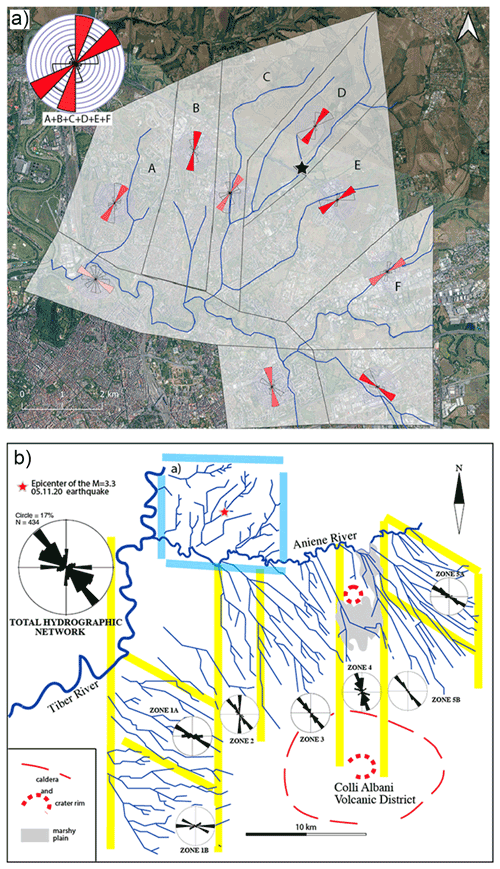
Figure 5(a) The result of the streambed direction analysis performed in this work within the hydrographic basin including the epicenter area of the 11 May event (pale blue borders in b) is compared with that performed in the southeastern Roman area, between the Tiber, the Aniene and the Colli Albani (b) (Marra, 2001). Yellow lines border the different sectors of the analyzed drainage basins. Analysis in the historical city center was hindered by the occurrence of a widespread anthropic cover. Basemap from QGIS QuickMapServices (available under Creative Commons Attribution-ShareAlike 3.0 licence (CC BY-SA) at https://plugins.qgis.org/plugins/quick_map_services/, last access: 23 July 2022).
5.3 Streambed analysis
In order to compare the results with previous analysis of the regional deformation pattern, a quantitative analysis of drainage trends has been performed in the discrete hydrographic basin located in the sector NE of the Tiber and Aniene confluence (Fig. 5a), within which the 11 May earthquake occurred.
The streambed direction analysis within the hydrographic basin including the epicenter area of the 11 May event was created by using the QGIS “Line Direction Histogram” plugin (Tveite, 2015) that visualizes the distribution of line segment directions as a rose diagram (weighted using the line segment lengths). The number of bins of direction which compose the rose diagram could be set, and in this work we used eight bins corresponding to the main cardinal directions. The tiles into which the area has been divided were identified according to the main directions of streambeds.
5.4 Drainage network anomalies and river profile analysis
Drainage network anomalies are one of the most useful morphotectonic indicators of active tectonics, and they are widely used as an effective tool to infer the possible control of fault activity on landscape and channels (see, for example, Boulton et al., 2014; Calzolari et al., 2016; Pavano et al., 2016; Kent et al., 2017; Bahrami, 2013). Integrated studies of possible active tectonic control on the geometry of the drainage network frequently include analysis of river longitudinal profiles, preferential orientation and alignments of channels, right-angle confluences, and fluvial elbows (Boulton et al., 2014; Pavano et al., 2016; Kent et al., 2017; Gioia et al., 2018). Indeed, river profile analysis is one of the most powerful tools for the identification of the transient state of a drainage network and recognition of knickpoints/knickzones, which represent valuable and effective morphotectonic markers of recent crustal deformation (Whipple and Tucker, 1999). Our approach combines the analysis of anomalies in drainage network geometry (i.e., preferential orientation and/or alignments of channels, fluvial elbows, right-angle confluences) with the identification of knickpoints/knickzones of tectonic origin in transient longitudinal river profiles. Such data have been used as morphotectonic evidence of active/recent tectonic deformation induced by the fault system responsible for the seismic activity of the study area.
River profile analysis has been carried out according to the methods and procedures developed by Wobus et al. (2006) and Forte and Whipple (2019) using a DEM with a spatial resolution of 10 m. Stream profile analysis is classically carried out by identifying knickpoints or knickzones along the river longitudinal profiles or by extracting a linear regression in a log–log slope–area graph, which allowed us to extrapolate the concavity index (the slope of the regression) and the steepness index (the y intercept, which is the projection of the best-fit line that intersects the y axis). Knickpoints or abrupt scarps of the longitudinal profiles can be related to tectonic- or eustatic-induced perturbations of ancient base levels, but their formation and migration can be also related to co-seismic fault ruptures or deformation induced by blind faults (Kirby and Whipple, 2012). In particular, the identification of fault-induced disturbance on channel profiles can be performed through the recognition of linear alignments of knickpoints/knickzones in channels with different sizes and orientations (Boulton et al., 2014; Kirby and Whipple, 2012).
In order to investigate the possible occurrence of fault-related knickpoints and river profile anomalies, we have investigated the river longitudinal profiles of the main channels of the study area through the identification and mapping of abrupt changes in river profile shape. Such data have been combined with the morphotectonic analysis of the spatial distribution of drainage network anomalies. Then, their spatial distribution has been used to infer the traces of possible tectonic lineaments of the study area.
6.1 Focal mechanism and re-location of the 11 May earthquake
The Ml 3.3 mainshock (11 May at 03:03 UTC) was followed over the next 2 d by only four small aftershocks with magnitudes ranging from 0.7 to 1.8 (Table 2). Thanks to the high station coverage we were able to determine all earthquake hypocenter depths with acceptable uncertainties. The average location errors are 0.14 km (horizontally) and 0.32 km (vertically) with a confidence level of 90 %. The mainshock hypocenter is at 9.6 km of depth, while the aftershock hypocenters are ranging from 5.0 to 11.2 km of depth (Fig. 4). The two largest aftershocks (magnitude Ml 1.8 and 1.4, respectively) have depths between 5.0 and 5.8 km and are located very close to the mainshock epicenter, while the two smallest aftershocks (both magnitude Ml 0.7) are located slightly towards the NW with respect to the mainshock epicenter, at 7.2 and 11.2 km of depth. These two aftershocks are clearly unrelated to the seismogenic structure responsible for the mainshock and are likely the effect of stress propagation to a contiguous fault.
We have computed the fault plane solution of the mainshock with the FPFIT code (Reasenberg and Oppenheimer, 1985). First-motion polarities are 57. The focal mechanism has a large strike-slip component (first nodal plane: strike 15, dip 85, rake −10). The T axis is oriented in a NE–SW direction according with the general “Antiapennine” (NE–SW) extension. Following some tectonic information of this area, the fault plane coincides with the NNE–SSW nodal plane of the solution which has a left-lateral strike-slip kinematics.
6.2 Statistical analysis of streambed directions in the epicenter area
Results of the streambed analysis in the small hydrographic basin where the epicenter of the 11 May earthquake occurred are summarized in Fig. 5a.
The streambeds in the eastern portion of the basin (discrete sectors D, E, F) concentrate around the NE–SW direction, which is the one expected based on the topographic gradient, perpendicular to the Aniene River course, towards which the catchment basin drains. In contrast, an abrupt rotation occurs in the western portion of the basin (discrete sectors A, B, C), where the streambeds are aligned along the NNE–SSW direction, parallel to the main watercourse of the Tiber River. Similarly to the results obtained in the southern area by Marra (2001), showing that the ca. N–S direction is a characteristic feature of the streambeds in this region which is clearly independent of the geographic and topographic control on the hydrographic network, we interpret the N–S lineaments as reflecting tectonic control on the streambeds exerted by fault activity in the analyzed basin. As has been remarked in previous works (e.g., Alfonsi et al., 1991; Faccenna et al., 1994a, 2008; Marra et al., 2004b), strike-slip, right-lateral N–S faults have been active repeatedly during the Pleistocene, up to historical times. Frepoli et al. (2010) have remarked on the direct relationship between the sectors characterized by the N–S direction of the streambeds and seismically active fault zones. It is worth noting that the 11 May earthquake epicenter occurs on the northern continuation of one such N–S zone (zone 2 in Fig. 5b).
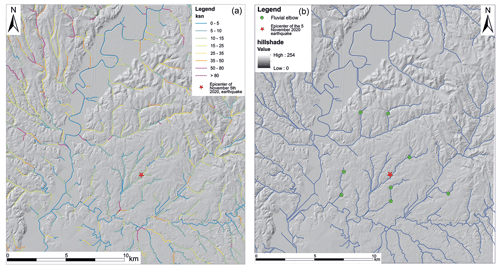
Figure 6(a) Hillshade of the study area and distribution of the normalized channel steepness index (ksn, θref = 0.45). (b) Drainage network of the study area and main planar anomalies of the fluvial net. Tectonic lineaments inferred by morphotectonic analysis are also shown. Published with a CC BY 4.0 license by Istituto Nazionale di Geofisica e Vulcanologia (INGV), available at: https://doi.org/10.13127/TINITALY/1.0.
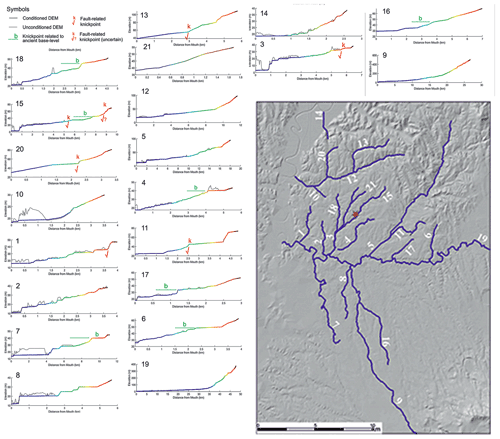
Figure 7Longitudinal profiles of the main channels of the study area (location and numbering in the main map) and interpretation of the knickpoints. published with a CC BY 4.0 license by Istituto Nazionale di Geofisica e Vulcanologia (INGV), available at: https://doi.org/10.13127/TINITALY/1.0.
6.3 Morphotectonic analysis of the drainage network: river profile analysis and drainage network anomalies
The analysis of longitudinal river profiles of the bedrock rivers is based on the stream power incision model (Whipple and Tucker, 1999; Wobus et al., 2006; Forte and Whipple, 2019) and has been carried out to evaluate the channel response to eustatic- and tectonic-induced processes. In a first step, we prepare a map of the normalized steepness index (ksn) with a reference concavity index of θref = 0.45 (Fig. 6a). The ksn map allowed us to perform a preliminary analysis of the spatial distribution of ksn values, which can be useful to individuate the sectors of the landscape featured by knickpoints and knickzones of tectonic origin. Moreover, a morphotectonic map showing the spatial distribution of fluvial elbows and anomalies in drainage network geometry was also introduced (Fig. 6b). Figure 7 shows the results of the analysis of the river profiles, which highlights how most of the channels deviate from the typical equilibrium shape of the longitudinal profiles. Longitudinal profiles are featured by the presence of knickpoints and knickzones, mainly in the central reach of the river profiles. These knickpoints appear to not be controlled by lithological contact and suggest a transient state of the fluvial net induced by tectonic perturbation or eustatic base-level variations. In particular, we detect the occurrence of convex zones or knickpoints related to past base levels, as testified by the presence of large “terraced surfaces” at altitudes ranging from 60 to 40 m a.s.l. (Fig. 7). Our analysis also reveals the occurrence of a cluster of knickpoints on the orographic right side of the Aniene River with different features than the previous ones. In fact, they can be classified as slope-break knickpoints (sensu Wobus et al., 2006; see also Kirby and Whipple, 2012) and are aligned along a NW–SE and N–S orientation. Such alignments, as well as the location of anomalous confluences and right-angle elbows of the drainage network, allowed us to infer the occurrence of the tectonic lineaments mapped in Fig. 8, which can be responsible for the recent tectonic activity that promoted the perturbation of the fluvial net.
Studies conducted during the last two decades on the structural-geological and seismotectonic setting of the Roman area have shown that the geometry of the hydrographic network reflects that of a set of buried faults (Marra, 1999, 2001; Frepoli et al., 2010). Considering the significant offsets affecting the Middle Pleistocene volcanic deposits in this area (e.g., Faccenna et al., 1994a, b; Marra, 2001) compared to the lack of strong events in the historical record, it is inferred that these faults are no longer active with the seismic intensity they had in the geological past. We conclude that they are reactivated under the effect of the stress field that currently acts in the upper crust and determines the genesis of low-magnitude earthquakes in this region.
In particular, it has been shown that the drainage network pattern and the distribution of river profile anomalies (i.e., fluvial elbows and knickpoint/knickzones) reflect the deformation field induced on the surface by the reactivation of these buried faults with a set of three preferential alignments.
- i.
The first displays a NW–SE “Apennine” direction (“a” in Fig. 9) which precisely reflects that of the large, dip-slip extensional faults that first created the Tyrrhenian Sea marine basins (Barberi et al., 1994) and later, in the Lower–Middle Pleistocene, the so-called “Tyrrhenian margin” (Fig. 2). This is a wide hilly or sub-flat area between the Apennine chain and the present coast, originated by the fault displacement and the “staircase” lowering of the mountain relief (Parotto and Praturlon, 1975). The direction of these faults also reflects the alignment of the volcanoes that developed in the Middle Pleistocene along the Tyrrhenian margin, following the rise of magmas mainly along the fractures in the earth's crust created by these tectonic structures (Locardi et al., 1977).
- ii.
The second set of lineaments has a direction from N–S to NNE–SSW (“b” in Fig. 9) and reflects that of even older faults, with a right-lateral strike-slip character, i.e., sub-vertical faults with right-hand horizontal movement (Alfonsi et al., 1991; Faccenna et al., 1994a). These faults are linked to the dismemberment of the Apennine chain in independent arcs due to the fragmentation of the “slab”, which is the “Adriatic” tectonic plate that subducted below the Apennine orogenetic chain (Malinverno and Ryan, 1986; Patacca and Scandone, 1989). However, these faults have been active into recent times (Faccenna et al., 2008; Marra et al., 2004b), probably due to the independent geodynamic mechanism that generated them, and are competing with the regime of forces that originated the extensional faults (Marra, 2001; Faccenna et al., 1996). We also know from the analysis of the focal mechanisms of local earthquakes that small N–S fault segments are currently reactivated with opposite movement (left-lateral), together with the “Apennine” dip-slip faults (Frepoli et al., 2010).
- iii.
Finally, a third set of lineaments has conjugated WNW–ESE and ENE–WSW directions (“c” and “c' ” in Fig. 9) and creates particular rhomboid “domains”. Within these discrete regions, the N–S direction (as in the case of the epicenter area of Rome's 11 May 2020 earthquake, Fig. 9) or the same WNW–ESE directions (zones 1A and 5A in Fig. 5b) may prevail. The origin of these domains is linked to the strike-slip faults and can be generated between two long, parallel N–S lineaments (Jones and Tanner, 1995). The characteristic of the strike-slip (transcurrent) faults is precisely that of being arranged in parallel with “en-echelon” geometry, that is, along stairway segments which can, however, locally have a lateral overlap between them (Sylvester, 1988). The en-echelon geometry characterizes the surface expression of faults that are continuous at depth (Sylvester, 1988) (examples “b' ” and “b” ” in Fig. 9).
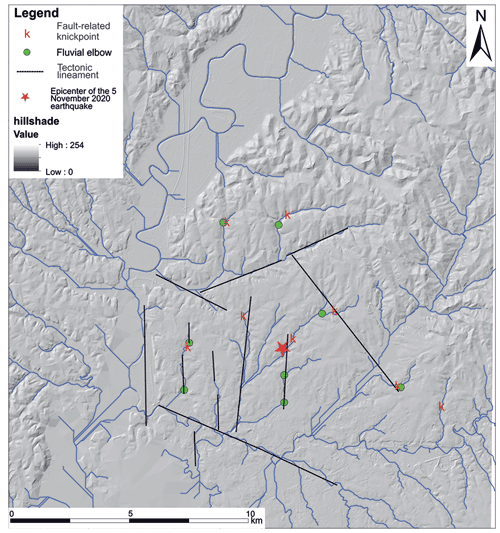
Figure 8Tectonic lineaments of the study area inferred by morphotectonic analyses and the spatial distribution of the main drainage network anomalies of the study area (i.e., fluvial elbow and knickpoints of river profiles). Hillshade was derived by the 10 m TINITALY DEM, published with a CC BY 4.0 license by Istituto Nazionale di Geofisica e Vulcanologia (INGV), available at: https://doi.org/10.13127/TINITALY/1.0.
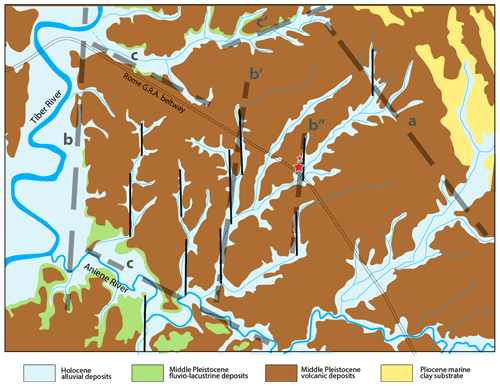
Figure 9Geo-morphostructural setting of the epicenter area. The thicker dashed lines represent the main buried faults inferred from the analysis of the hydrographic network, with the exception of the “a” fault, interpreted on the basis of the presence of a structural high to the NE, represented by outcrops of Pliocene sediments. A fourth set of NE–SW lineaments is likely originated by the topographic gradient in this area and is not highlighted as a potential structural control. The thin, solid lines represent the superficial expression of the deformation linked to faults that are continuous at depth (b', b”), evidenced by straight tracts of the riverbeds. One of these deep NNE–SSW faults is the one that generated the 11 May earthquake, as the focal mechanism of this event suggests.
The analysis of the hydrographic network in the epicenter area of the 11 May 2020 earthquake shows a relative maximum concentration of the streambed in the NNE–SSW direction: some of such rectilinear tracts, arranged with en-echelon geometry, are highlighted in Fig. 5. We interpret these features as the surface expression of buried NNE–SSW strike-slip faults. Indeed, the focal mechanism and aftershock alignment reveal that one of these buried ∼ N–S faults reactivated with left-lateral movement on the occasion of the 11 May 2020 earthquake. Effectively, tectonically sensitive geomorphic analyses revealed the occurrence of a cluster of knickpoints on the right side of the Aniene River that can be classified as slope-break knickpoints and are aligned along a NW–SE and N–S orientation. Such a fluvial net perturbation corroborates the hypothesis of recent tectonic activity affecting the study area along those faults.
When we consider the multitude of lineaments that are present at a wider and at a smaller scale in this region (e.g., Figs. 2 and 9, respectively), we realize the extreme fragmentation deriving from the intricate network of genetically different faults. Such fragmentation results in a number of small fault segments with respect to the original long fault lines generated under the competitive tectonic regimes that affected this region during Pleistocene times. We remark that such high fragmentation is mainly provided by an en-echelon system of ∼ N–S strike-slip faults which have crustal continuity, therefore hindering the lateral continuity of the NW–SE trending faults, which represent the most favorably oriented fault system with respect to the present-day NE–SW extensional regime.
Small fault planes and a weaker tectonic regime explain the occurrence of moderate seismicity and provide a likely explanation for the inhabitants of Rome of the reason why they should not expect that a large earthquake will affect the city.
All data generated or analyzed during this study are included in this published article.
FM was responsible for conceptualization, methodology, validation, investigation, writing the original draft and supervision. AF, DG, MS and AT were responsible for methodology, validation, investigation, data curation and writing the original draft. MB, GDL and ML were responsible for methodology, validation, investigation, data curation, writing, reviewing and editing.
The contact author has declared that none of the authors has any competing interests.
Publisher’s note: Copernicus Publications remains neutral with regard to jurisdictional claims in published maps and institutional affiliations.
This paper was edited by Filippos Vallianatos and reviewed by two anonymous referees.
Acocella, V. and Funiciello, R.: Transverse systems along the extensional Tyrrhenian margin of central Italy and their influence on volcanism, Tectonics, 25, TC2003, https://doi.org/10.1029/2005tc001845, 2006.
Alfonsi, L., Funiciello, R., Mattei, M., Girotti, O., Maiorani, A., Preite Martinez, M., Trudu, C., and Turi, B.: Structural and geochemical features of the Sabina strike-slip fault (Central Apennines), B. Soc. Geol. Ital., 110, 217–230, 1991.
Amato, A. and Chiarabba, C.: Earthquake occurrence and crustal structure, in: The Volcano of the Alban Hills, edited by: Trigila, R., Univ. degli Studi di Roma “La Sapienza”, Rome, La Sapienza University of Rome, Italy, 193–211, 1995.
Amato, A., Alessandrini, B., Cimini, G. B., Frepoli, A., and Selvaggi, G.: Active and remnant subducted slabs beneath Italy: evidence from seismic tomography and seismicity, Ann. Geofis., 36, 201–214, 1993.
Bahrami, S.: Analyzing the drainage system anomaly of zagros basins: Implications for active tectonics, Tectonophysics, 608, 914–928, 2013.
Barberi, F., Buonasorte, G., Cioni, R., Fiordelisi, A., Foresi, L., Iaccarino, S., Laurenzi, M. A., Sbrana, A., Vernia, L., and Villa, I. M.: Plio-Pleistocene geological evolution of the geothermal area of Tuscany and Latium, Mem. Descr. Carta Geol. Ital., 49, 77–134, 1994.
Basili, A., Cantore, L., Cocco, M., Frepoli, A., Margheriti, L., Nostro, C., and Selvaggi, G.: The June 12, 1995 microearthquake sequence in the city of Rome, Ann. Geofis., 39, 1167–1175, 1996.
Boulton, S. J., Stokes, M., and Mather, A. E.: Transient fluvial incision as an indicator of active faulting and Plio-Quaternary uplift of the Moroccan High Atlas, Tectonophysics, 633, 16–33, https://doi.org/10.1016/j.tecto.2014.06.032, 2014.
Calzolari, G., Della Seta, M., Rossetti, F., Nozaem, R., Vignaroli, G., Cosentino, D., and Faccenna, F.: Geomorphic signal of active faulting at the northern edge of Lut Block: Insights on the kinematic scenario of Central Iran, Tectonics, 35, 76–102, https://doi.org/10.1002/2015TC003869, 2016.
Caputo, C., Ciccacci, S., De Rita, D., Fredi, P., Lupia Palmieri, E., and Salvini, F.: Drainage pattern and tectonics in some volcanic areas of Latium (Italy), Geologica Romana, 29, 1–13, 1993.
Chatelain, J. L.: Etude fine de la sismicité en zone de collision continentale à l'aide d'un réseau de stations portables: la region Hindu-Kush-Pamir, Thèse de 3 éme cycle, Univ. Paul Sabatier, Toulouse, 1978.
Ciccacci, S., Fredi, P., Lupia Palmieri, E., and Salvini, F.: An approach to the quantitative analysis of the relations between drainage pattern and fracture trend, in: International Geomorphology 1986, edited by: Gardiner, V., Proceedings of the First International Conference on Geomorphology, Part II, John Wiley and Sons Ltd, Chichester, 49–68, 1987.
Del Monte, M., D'Orefice, M., Luberti, G. M., Marini, R., Pica, A., and Vergari, F.: Geomorphological classification of urban landscapes: the case study of Rome (Italy), J. Maps, 12, 178–189, https://doi.org/10.1080/17445647.2016.1187977, 2016.
De Luca, G., Cattaneo, M., Monachesi, G., and Amato, A.: Seismicity in central and northern Apennines integrating the Italian national and regional networks, Tectonophysics, 476, 121–135, https://doi.org/10.1016/j.tecto.2008.11.032, 2009.
Faccenna, C., Funiciello, R., and Mattei, M.: Late Pleistocene N–S shear zones along the Latium Tyrrhenian margin: structural characters and volcanological implications, Bollettino di Geofisica Teorica Applicata, 36, 507–522, 1994a.
Faccenna, C., Funiciello, R., Montone, P., Parotto, M., and Voltaggio, M.: An example of late Pleistocene strike-slip tectonics: the Acque Albule basin (Tivoli, Latium), Mem. Descr. d. Carta Geol. d'It., 49, 37–50, 1994b.
Faccenna, C., Davy, P., Brun, J. P., Funiciello, R., Giardini, D., Mattei, M., and Nalpas, T.: The dynamics of back-arc extension: an experimental approach to the opening ofthe Tyrrhenian Sea, Geophys. J. Int., 126, 781–795, 1996.
Faccenna, C., Soligo, M., Billi, A., De Filippis, L., Funiciello, R., Rossetti, C., and Tucciemei, P.: Late Pleistocene depositional cycles of the Lapis Tiburtinus travertine (Tivoli, Central Italy): Possible influence of climate and fault activity, Global Planet. Change, 63, 299–308, https://doi.org/10.1016/j.gloplacha.2008.06.006, 2008.
Forte, A. M. and Whipple, K. X.: Short communication: The Topographic Analysis Kit (TAK) for TopoToolbox, Earth Surf. Dynam., 7, 87–95, https://doi.org/10.5194/esurf-7-87-2019, 2019.
Frepoli, A. and Amato, A.: Contemporaneous extension and compression in the northern Apennines from earthquake fault-plane solutions, Geophys. J. Int., 129, 368–388, 1997.
Frepoli, A., Marra, F., Maggi, C., Marchetti, A., Nardi, A., Pagliuca, N. M., and Pirro, M.: Seismicity, seismogenic structures and crustal stress field in the greater area of Rome (Central Italy), J. Geophys. Res., 115, B12303, https://doi.org/10.1029/2009JB006322, 2010.
Frepoli, A., Cimini, G.B., De Gori, P., De Luca, G., Marchetti, A., Monna, S., Montuori, C., Pagliuca, N.: Seismic sequences and swarms in the Latium-Abruzzo-Molise Apennines (central Italy): new observations and analysis from a dense monitoring of the recent activity, Tectonophysics, 712–713, 312–329, https://doi.org/10.1016/j.tecto.2017.05.026, 2017.
Galli, P. A. C. and Molin, D.: Beyond the damage threshold: the historic earthquakes of Rome, B. Earthquake Eng., 12, 1277–1306, https://doi.org/10.1007/s10518-012-9409-0, 2014.
Gaeta, M., Freda, C., Marra, F., Arienzo, I., Gozzi, F., Jicha, B., Di and Rocco, T.: Paleozoic metasomatism at the origin of Mediterranean ultrapotassic magmas: constraints from time-dependent geochemistry of Colli Albani volcanic products (Central Italy), Lithos, 244, 151–164, 2016.
Gioia, D., Schiattarella, M., and Giano, S.: Right-Angle Pattern of Minor Fluvial Networks from the Ionian Terraced Belt, Southern Italy: Passive Structural Control or Foreland Bending?, Geosciences, 8, 331, https://doi.org/10.3390/geosciences8090331, 2018.
Guidoboni, E., Ferrari, G., Mariotti, D., Comastri, A., Tarabusi, G., Sgattoni, G., and Valensise, G.: CFTI5Med, Catalogo dei Forti Terremoti in Italia (461 a.C.–1997) e nell'area Mediterranea (760 a.C.–1500), Istituto Nazionale di Geofisica e Vulcanologia (INGV), http://storing.ingv.it/cfti/cfti5 (last access: 23 July 2022), 2018.
Holland, J. H.: Adaptation in Natural and artificial systems, University of Michigan Press, Ann Arbor, 1975.
Horvath, F. and Berckhemer, H.: Mediterranean back arc basins, in: Alpine Mediterranean Geodynamics, 145–175, edited by: Berckhemer, H. and Hsu, K. J., Geodyn. Ser., 7, American Geophys. Un., Whashington, D.C., ISBN 978-1-118-67024-8, 1982.
Jones, R. R. and Tanner, P. W. G.: Strain partitioning in transpression zones, J. Struct. Geol., 17, 793–802, 1995.
Kent, E., Boulton, S. J., Whittaker, A. C., Stewart, I. S., Cihat and Alçiçek, M.: Normal fault growth and linkage in the Gediz (Alaşehir) Graben, Western Turkey, revealed by transient river long-profiles and slope-break knickpoints, Earth Surf. Proc. Land., 42, 836–352, https://doi.org/10.1002/esp.4049, 2017.
Kirby, E. and Whipple, K. X.: Expression of active tectonics in erosional landscapes, J. Struct. Geol., 44, 54–75, https://doi.org/10.1016/j.jsg.2012.07.009, 2012.
Lahr, J. C.: HYPOELLIPSE/Version 2.0: a computer program for determining local earthquake hypocentral parameters, magnitude and first-motion pattern, U.S. Geol. Surv. Open-file Report, 95, 89–116, https://doi.org/10.3133/ofr89116, 1989.
Locardi, E., Lombardi, G., Funiciello, R., Parotto, M.: The Main volcanic groups of Latium (Italy): relations between structural evolution and petrogenesis, Geologica Romana, 15, 279–300, 1977.
Lucente, F. P. and Speranza, F.: Belt bending driven by lateral bending of subducting lithospheric slab: geophysical evidences from the northern Apennines (Italy), Tectonophysics, 337, 53–64, 2001.
Macka, Z.: Structural control on drainage network orientation an example from the Loucka drainage basin, SE margin of the Bohemian Massif (S Moravia, Czech Rep.), Landform Analysis, 4, 109–117, 2003.
Malinverno, A. and Ryan, W. B. F.: Extension in the Tyrrhenian sea and shortening in the Apennines as results of arc migration driven by sinking of the lithosphere, Tectonics, 5, 227–245, 1986.
Mariucci, M. T., Amato, A., and Montone, P.: Recent tectonic evolution and present stress in the Northern Apennines (Italy), Tectonics, 18, 108–118, 1999.
Marra, F.: Low-magnitude earthquakes in Rome: structural interpretation and implications for local stress-field, Geophys. J. Int., 138, 231–243, 1999.
Marra, F.: Strike-slip faulting and block rotation: A possible triggering mechanism for lava flows in the Alban Hills?, J. Struct. Geol., 23, 129–141, 2001.
Marra, F., Taddeucci, J., Freda, C., Marzocchi, W., and Scarlato, P.: Recurrence of volcanic activity along the Roman Comagmatic Province (Tyrrhenian margin of Italy) and its tectonic significance, Tectonics, 23, TC4013, https://doi.org/10.1029/2003TC001600, 2004a.
Marra, F., Montone, P., Pirro, M., and Boschi, E.: Evidence of Active Tectonics on a Roman Aqueduct System (II–III Century A.D.) near Rome, Italy, J. Struct. Geol., 26, 679–690, 2004b.
Marra F., Karner, D. B., Freda, C., Gaeta, M., and Renne, P. R.: Large mafic eruptions at the Alban Hills Volcanic District (Central Italy): chronostratigraphy, petrography and eruptive behavior, J. Volcanol. Geoth. Res., 179, 217–232, https://doi.org/10.1016/j.jvolgeores.2008.11.009, 2009.
Marra, F., Sottili, G., Gaeta, M., Giaccio, B., Jicha, B., Masotta M., and Palladino, D.: Major explosive activity in the Sabatini Volcanic District (central Italy) over the 800–390 ka interval: geochronological – geochemical overview and tephrostratigraphic implications, Quaternary Sci. Rev., 94, 74–101, https://doi.org/10.1016/j.quascirev.2014.04.010, 2014.
Marra, F., Florindo, F., Anzidei, M., and Sepe, V.: Paleo-surfaces of glacio-eustatically forced aggradational successions in the coastal area of Rome: assessing interplay between tectonics and sea-level during the last ten interglacials, Quaternary Sci. Rev., 148, 85–100, https://doi.org/10.1016/j.quascirev.2016.07.003, 2016.
Molin, D. and Rossi, A.: Effetti prodotti in Roma dai terremoti del 1703, in: Settecento abruzzese. Eventi sismici, mutamenti economico-sociali e ricerca storiografica, edited by: Colapietra, R., Marinangeli, G., and Muzzi, P., 69–106, ISBN 8888676414, 2004.
Montone, P. and Mariucci, M. T.: The new release of the Italian contemporary stress map, Geophys. J. Int., 205, 1525–1531, https://doi.org/10.1093/gji/ggw100, 2016.
Montone, P., Amato, A., Chiarabba, C., Buonasorte, G., and Fiordelisi, A.: Evidence of active extension in Quaternary volcanoes of Central Italy from breakout analysis and seismicity, Geophys. Res. Lett., 22, 1909–1912, 1995.
Patacca, E. and Scandone, P.: Post-Tortonian mountain building in the Apennines. The role of the passive sinking of a relic lithospheric slab, in: The Lithosphere in Italy, edited by: Boriani, A., Bonafede, M., Piccardo, G. B., and Vai, G. B., Advances in Earth Science Research, It. Nat. Comm. Int. Lith. Progr., Mid-term Conf., Rome, 5–6 May 1987, Atti Conv. Lincei, 80, 157–176, 1989.
Parotto, M. and Praturlon, A.: Geological summary of the Central Appenines, in: Structural Model of Italy, edited by: Ogniben, L., Parotto, M., and Praturlon, A., Quad. Ric. Scient., 90, 257–311, 1975.
Pavano, F., Pazzaglia, F. J., and Catalano, S.: Knickpoints as geomorphic markers of active tectonics: A case study from northeastern Sicily (southern Italy), Lithosphere, 8, 633–648, https://doi.org/10.1130/L577.1, 2016.
Peccerillo, A.: Cenozoic Volcanism in the Tyrrhenian Sea Region, S. IAVCEI, Barcelona, Springer, ISBN 978-3-319-42491-0, 2017.
Reasenberg, P. and Oppenheimer, D.: FPFIT, FPPLOT and FPPAGE: FORTRAN computer programs for calculating and displaying earthquake fault plane solutions, USGS Open-file Report, 85–739, https://doi.org/10.3133/ofr85739 1985.
Reutter, K. J., Giese, P., and Closs, H.: Lithospheric split in the descending plate: observation from the Northern apennines, Tectonophysics, 64, T1–T9, 1980.
Rovida, A., Locati, M., Camassi, R., Lolli, B., and Gasperini, P.: The Italian earthquake catalogue CPTI15, B. Earthqu. Eng., 18, 2953–2984, https://doi.org/10.1007/s10518-020-00818-y, 2020.
Sambridge, M. and Gallagher, K.: Earthquake hypocenter location using genetic algorithms, B. Seismol. Soc. Am., 83, 1467–1491, 1993.
Selvaggi, G., and Amato, A.: Subcrustal earthquakes in the Northern Apennines (Italy): evidence for a still active subduction?, Geophys. Res. Lett., 19, 2127–2130, 1992.
Serri, G.: Neogene-Quaternary magmatic activity and its geodynamic implications in the Central Mediterranean region, Ann. Geofis., 3, 681–703, 1997.
Serri, G., Innocenti, F., and Manetti, P.: Geochemical and Petrological evidence of the subduction of delaminated Adriatic continental lithosphere in the genesis of the Neogene-Quaternary magmatism of Central Italy, Tectonophysics, 223, 117–147, 1993.
Sylvester, A. G.: Strike-slip faults, GSA Bulletin, 100, 1666–1703, https://doi.org/10.1130/0016-7606(1988)100<1666:SSF>2.3.CO;2, 1988.
Tapponnier, P.: Evolution tectonique du systeme alpin en Mediterranee; poinconnement et ecrasement rigide-plastique, B. Soc. Géol. Fr., 3, 437–460, https://doi.org/10.2113/gssgfbull.S7-XIX.3.437, 1977.
Tertulliani, A. and Riguzzi, F.: Earthquakes in Rome during the past one hundred years, Ann. Geofis., 38, 591–606, 1995.
Tertulliani, A., Graziani, L., and Esposito, A.: How historical seismology can benefit from bureaucracy: the case of the “Lettere Patenti” of the city of Rome in 1703, Seismol. Res. Lett., 91, 2511–2519, https://doi.org/10.1785/0220200046, 2020.
Trasatti, E., Marra, F., Polcari, M., Etiope, G., Ciotoli, G., Darrah, T., Tedesco, D., Florindo, F., and Ventura, G.: Coeval uplift and subsidence reveal magma recharging near Rome, Geochem. Geophy. Geosy., 19 1484–1498, https://doi.org/10.1029/2017GC007303, 2018.
Tveite, H.: The QGIS Line Direction Histogram Plugin, http://plugins.qgis.org/plugins/LineDirectionHistogram/ (last access: 23 July 2022), 2015.
Whipple, K. X. and Tucker, G. E.: Dynamics of the stream-power river incision model: Implications for height limits of mountain ranges, landscape response timescales, and research needs, J. Geophys. Res.-Sol. Ea., 104, 17661–17674, https://doi.org/10.1029/1999JB900120, 1999.
Wobus, C., Whipple, K. X., Kirby, E., Snyder, N., Johnson, J., Spyropolou, K., Crosby, B., and Sheehan, D.: Tectonics from topography: Procedures, promise, and pitfalls, Special Paper of the Geological Society of America, 55–74, https://doi.org/10.1130/2006.2398(04), 2006.