the Creative Commons Attribution 4.0 License.
the Creative Commons Attribution 4.0 License.
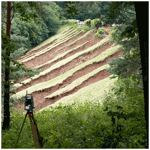
More than heavy rain turning into fast-flowing water – a landscape perspective on the 2021 Eifel floods
Rainer Bell
Ugur Ozturk
Kristen L. Cook
Christoff Andermann
Alexander R. Beer
Bodo Damm
Ana Lucia
Felix S. Fauer
Katrin M. Nissen
Tobias Sieg
Annegret H. Thieken
Rapidly evolving floods are rare but powerful drivers of landscape reorganisation that have severe and long-lasting impacts on both the functions of a landscape’s subsystems and the affected society. The July 2021 flood that particularly hit several river catchments of the Eifel region in western Germany and Belgium was a drastic example. While media and scientists highlighted the meteorological and hydrological aspects of this flood, it was not just the rising water levels in the main valleys that posed a hazard, caused damage, and drove environmental reorganisation. Instead, the concurrent coupling of landscape elements and the wood, sediment, and debris carried by the fast-flowing water made this flood so devastating and difficult to predict. Because more intense floods are able to interact with more landscape components, they at times reveal rare non-linear feedbacks, which may be hidden during smaller events due to their high thresholds of initiation. Here, we briefly review the boundary conditions of the 14–15 July 2021 flood and discuss the emerging features that made this event different from previous floods. We identify hillslope processes, aspects of debris mobilisation, the legacy of sustained human land use, and emerging process connections and feedbacks as critical non-hydrological dimensions of the flood. With this landscape scale perspective, we develop requirements for improved future event anticipation, mitigation, and fundamental system understanding.
- Article
(10300 KB) - Full-text XML
- BibTeX
- EndNote
The 14–15 July 2021 flood in western Germany as well as parts of Belgium and the Netherlands revealed the unpreparedness of societies, policymakers, and scientists across many dimensions. The anticipated precipitation amounts had been communicated several days ahead; hydrological models and numerous stream gauges were in place; and information chains and authority responsibilities were implemented. Yet the flood, which hit several catchments that originate in the Eifel (Fig 1), developed into a massive hazard event that propagated downstream for long distances and persisted for many hours. Meanwhile, and evident from media reports focussing on the preceding rain event and the evolution of the flood, mitigation efforts remained insufficient; the actual flood wave was underpredicted; and emergency activities failed to prevent a disaster that led to 184 fatalities and 2 missing persons (as of 24 November 2021) in Germany alone (Thieken et al., 2021). This unexpectedly high toll is the highest in Germany for the past 6 decades.
The meteorological driver of the crisis was a cyclone named “Bernd” (Schneider and Gebauer, 2021). It travelled from the North Atlantic via France towards central Europe. Over western Germany, its propagation speed was slowed down by an anticyclone over eastern Europe, causing almost stationary precipitation over the Eifel region from 12 to 15 July, releasing 115 mm rain in 72 h on average in for example the Ahr catchment (Junghänel et al., 2021), with a maximum value of 157 mm on 14 July at the DWD (Deutscher Wetterdienst) station Köln-Stammheim. The soils in the entire region were already mostly saturated due to frequent previous rain events, which is expressed by an average free storage capacity <70 mm within the soils' top 60 cm as well as an average soil field capacity of 80 %–100 % (DWD-Agrowetter, 2022). The meteorological situation was properly forecasted days in advance by several weather prediction models (DWD, 2021; Schneider and Gebauer, 2021). The discrepancy between the accuracy of the meteorological forecasts and the shortcomings of flood hazard forecasting and communication reveals some of the challenges involved in anticipating the impacts of extreme events.
The next element of the evolutionary trajectory of the crisis was the surface runoff of excess rainfall that was not able to infiltrate into the ground and hence triggered several non-linear processes, positive feedbacks, and process connections (Dietze and Ozturk, 2021). Altogether, these dynamics amplified the impact of the flood on the landscape, particularly in the anthropogenic realm. To understand these dynamics, it is necessary to first examine the different landscape elements activated by the event and then to explore their modes of interplay. The flood hit several European countries. In Germany particularly, it impacted two geomorphically distinct regions: the Eifel with the Ahr valley to the south and the Lower Rhine Bay with the Erft catchment to the north. The Eifel is a typical low mountain range with steep, deeply incised valleys (see Fig. 1 for the slope signature). These valleys cut through numerous Paleozoic lithologies with varying degrees of fracturing, crack orientation, and ground permeability, which impose a pronounced predisposition to gravitational mass movement (Damm et al., 2010). In general, hillslopes are covered by Pleistocene, 1–3 m thick periglacial cover beds that are largely unstable due to the specific grain-size distribution, sensitive to water supply, and frequently incorporated in landslides (Bell, 2007; Damm et al., 2013). Rockfall-dominated cliffs are developed on steep slopes. In contrast, the Lower Rhine Bay region including the Erft catchment is an area of subsidence with smooth topography, filled by highly permeable Cenozoic sediments with a widespread cover of Quaternary loess deposits. There is no susceptibility to gravitational mass movement in this landscape, except for steep landforms that predominantly result from man-made construction (e.g. road escarpments, waste dumps, open-pit mining; cf. Fig 1). Both regions hold a long legacy of human land use.
Although the official data collection on economic flood impacts in Germany’s federal states of North Rhine-Westphalia and Rhineland-Palatinate is ongoing; current estimates point to EUR 33 billion in damage to private households, infrastructure, forestry, and agriculture as well as viticulture enterprises (Fekete and Sandholz, 2021). In Germany, the Ahr valley was hit hardest: 62 out of 75 bridges were destroyed, and almost all wineries were heavily affected (BMI, 2021). In Rhineland-Palatinate at least 65 000 people were directly affected by the event. A total of 135 people lost their lives; at least 766 people were injured; and 2 are still missing (Schmid-Johannsen et al., 2021). However, societal impacts occurred not only during the event itself. Months later, inner cities remain severely damaged, and commercial and gastronomy businesses are still heavily disrupted. Their reopening depends on the timely and simultaneous restoration of key infrastructure like electricity, telecommunications, water, and sewage. Disruption also affects schools and childcare facilities in some places, putting another burden on affected families. Physiological illnesses as well as psychological impacts on people who lost their homes, relatives, and friends during the flood represent a long-term legacy of the flood event beyond the areas actually impacted by the flood. Former floods in Germany revealed that a devastating experience preoccupied affected people for years (Thieken et al., 2016). Therefore, socio-psychological support is a key to recovery.
Based on empirical field studies during, immediately after, and over several weeks after the flood, we propose four critical non-hydraulic dimensions of flood-related processes. We present examples for each of these dimensions and discuss consequences of their interaction. These representative case studies form the basis of our synthesis of requirements to improve mitigation efforts for future events.
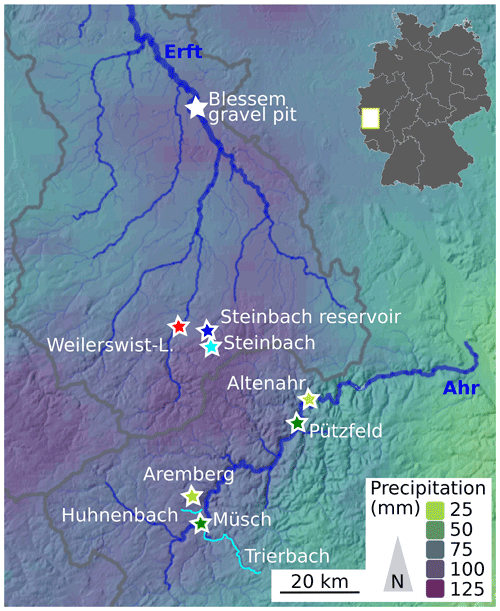
Figure 1Map of the affected area with 3 d precipitation accumulated for 12–15 July 2021 from RADOLAN data (Radargestützte Niederschlagsanalyse und -vorhersage; CDC, 2022). Stars indicate locations of pictures of other figures in this article. Light-blue lines depict rivers of special interest mentioned in the text. Background map shows two of the most affected river systems, Erft and Ahr (line width indicating stream order), on top of a hillshade and slope map. Inset shows the location of the map within Germany.
In this text, we decisively pursue a descriptive approach, focussing on generalised implications of systematic findings during field mapping campaigns carried out early after the flood event. There are several reasons for this approach. The amount and quality of data during the flood, such as gauge data, satellite imagery, and on-site instrumental data, are limited due to abundant cloud cover. Likewise, post-flood remote sensing data (BBK-DLR, 2022) is available for the main valley sections but not for headwater systems, where the flood gained its momentum and non-linearity (Dietze and Ozturk, 2021). Finally, model results that are currently produced do not capture the non-hydrodynamic dimension of the flood, while high-resolution 3D terrain information, essential for quantitative evaluation of erosion and deposition patterns, is currently still being produced (Bell et al., 2022; Wenzel et al., 2022).
Nevertheless, we are able to constrain the antecedent conditions; driver mechanisms; and, to some extent, the impacts of the flood event based on instrumental data. Spatially resolved precipitation information (Weigl and Winterrath, 2009; CDC, 2022) such as 1 km2 gridded hourly data is stacked as 72 h sums throughout the main event duration (12–15 July 2021). In addition, instrumental station data (CDC, 2022) of representative sites were used to characterise the severity of the rain event. We fitted intensity–duration–frequency (IDF) curves to historical annual maximum precipitation measurements. The IDF model was fitted with the extended consistent quantile estimation method (Fauer et al., 2021) and evaluated for the DWD station Weilerswist-Lommersum (Fig. 2).
Flood-affected areas and the surface change due to erosion and deposition of sediment and large woody debris were inspected by analysing aerial RGB (red–green–blue) imagery. The material was collected 1 d after the flood and allowed for a comparison of emerging surface features with imagery from months before the event (BBK-DLR, 2022). However, the availability and format of the data (web service) only allowed for non-quantified, descriptive studies.
Core information about the flood event is based on extensive and multi-temporal field mapping campaigns throughout the wider Eifel region, decisively including headwater regions and their coupling with tributaries of the Ahr River. Surveys of flood water marks, erosional features along channels and hillslopes, deposited sediment bodies caused by ponding and hydrodynamic ejection of bed material, and large woody debris accumulations were carried out 1 d after the flood and repeatedly within 2–3 weeks and 2–4 months. Surveys included the documentation of the type of feature as denoted above, information on the location and size, and a description of the geomorphic process regime responsible for its creation. The material presented in this text is limited to representative examples because a quantitative regionalisation needs to be based on post-event high-resolution terrain information (see above).
3.1 Hydrometeorological dimension
According to Fig. 1 based on radar and station observations (RADOLAN data set; Weigl and Winterrath, 2009) the precipitation amount for the 72 h period of 12–15 July at Weilerswist-Lommersum is similar to the amount observed in Blessem (Fig. 1). Moreover, the time series at the selected station is sufficiently long for the calculation of robust IDF curves (daily precipitation is available since 1905, as well as hourly precipitation since 2004). The analysis confirms the unusual extreme character of the precipitation amounts during the July event (black lines and crosses in Fig. 2). Sub-daily as well as daily and multi-day amounts exceeded the values corresponding to a 500-year return period determined under the assumption of stationary climate conditions.
3.2 Hillslope dimension
In typical scenarios, hillslope runoff delivers unconcentrated Hortonian or saturation overland flow to the streams, where discharge can accumulate and build up deeper and faster hydrographs. For the July 2021 flood, during post-event mapping campaigns we witnessed several occasions where ephemeral headwater drainages had turned into streams, conveying overland flow some decimetres deep (Fig. 3a). These local phenomena allowed overland flow velocity to increase by 1 to 2 orders of magnitude (Dietze and Ozturk, 2021), which was further amplified by the effects of infrastructure (see Sect. 2.3), such as forest tracks with rain ditches running alongside. While the tracks perpendicular to slope aspect were extensive runoff collectors (evidenced by systematic high water marks throughout mapped regions), the ditches efficiently conveyed collected water to release spots that acted as new gully heads (based on freshly developed erosional features). Once such gullies had formed, it is physically plausible that a positive feedback loop was implemented (Molina et al., 2009; Anderson and Anderson, 2010), leading to increasingly faster and more erosive discharge towards the valley bottoms. Hence, during the flood, concurrent landscape reorganisation, namely gullying by a concentration of overland flow amplified by infrastructure, most likely played a key role in changing the drainage efficiency. This spatial feedback is not accounted for in commonly applied hydraulic models.
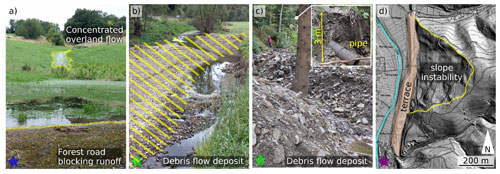
Figure 3Landscape features emerging from the flood. For locations of these pictures, see star signatures in Fig. 1. (a) Focussed discharge along the hillslope causing deep and fast flow and thus efficient drainage in the background. However, the provided water is not routed downslope in the foreground but ponded by infrastructure and released at selected spots with increased erosive stream power. (b) Deposition area of the debris flow shown in panel (c), injecting massive debris into the main channel (Trierbach), temporally blocking the stream and causing severe reorganisation of the hydraulic geometry. (c) Lateral deposits of the debris flow at the end of the valley-confined section. Inset shows upstream knickpoint formed by overspill and erosion of clogged drainage pipe (50 cm diameter). (d) Old slope instability (yellow line) above a 20 m high engineered terrace with industrial infrastructure on it. The terrace just east of the town of Antweiler had been undercut by the Ahr River during the flood.
Hillslopes contributed material not only through unconcentrated overland flow and focussed fluvial processes but also via gravitational mass wasting, most importantly in the form of debris flows and shallow and deep-seated landslides. During field mapping campaigns immediately after the event, we documented numerous debris flows emerging from hillslopes. These debris flows altered the hillslopes by erosion of soil and vegetation and severely impacted the channels that drain the valleys. They injected coarse particles and woody debris into the channels, which had a series of consequences. In many cases, the channel thalweg was displaced, or the entire trunk river was relocated within the flood plain; the local gradient was changed; and in some cases the entire flow was blocked at least temporarily (Fig. 3b). These abrupt changes have follow-on effects on channel morphology, for example bed armouring due to sediment sizes exceeding transport capacity, and the excavation of the bed including bedrock downstream, with enhanced capacity of future bedload transport and potential knickpoint migration upstream (Berger et al., 2011; Hu et al., 2021). Most of the mapped debris flow source points were locations where excess water was able to enter a debris flow channel, ultimately triggering this mass-wasting process. In most cases there were ditch-lined roads that collected and delivered the water. In addition to excess water, the investigated debris flows had at least one location in their contributing area that served as a massive source of mobile material. For example, the debris flow in Fig. 3c mobilised large amounts of material downstream of a 4–5 m high knickpoint, which formed by the overflow of a manufactured forest track crossing the channel. This artificial dam had a 50 cm drainage tube that quickly got clogged, causing backfilling and finally overtopping by the flood water. The high energy release at the resulting waterfall base caused erosion of the surrounding slope sections and the channel bed, sourcing rock material and trees into the debris channel and then into the main channel, the Trierbach, downstream (Fig. 1). Evidently, there were several previous debris flow deposits visible in the eroded bank of the Trierbach, indicating that the entering debris channel had been active several times in the past.
The landsliding component of hillslope material contribution had a series of triggers, mechanisms, and time lags to the precipitation phase. Post-flood mapping revealed numerous shallow landslides that were not related to fluvial undercutting as a trigger but were located on steep concave slope sections, hence at preferentially wetter slope positions (Giuseppe et al., 2021). This suggests that their activity was triggered by excess precipitation before and during the rainfall event. These features typically had a spatial extent of a few to a few tens of metres and were in most cases not connected to a channel. In contrast, there were also numerous river banks on the outer side of river bends that showed significant undercutting and, consequently, slope failures (Ozturk et al., 2018). These well-connected landscape elements were able to deliver sediment and woody debris directly to the channel (Fig. 4a). Such slope instabilities ranged in length from a few metres to features that have affected significant parts of valley hillslopes. In some cases, entire hillslopes with older instabilities were undercut (Fig. 3d) and might become reactivated subsequently. One such example of a river meander bend is depicted in Fig. 4b, where a 100 m long and 16 m high rock face was stripped of its debris apron as the Ahr River level rose by about 5 m above the current water level (cf. the flood impact scar in Fig. 4b). Subsequent visits to the site revealed traces of slope movement, such as extending cracks in a paved road crossing just above the rock slope. It is unclear how increased soil moisture during the winter period (Dietze et al., 2020) will affect the transient activity of this rock slide. Hence, further close monitoring of the slope instability is required to anticipate its failure and the potential for subsequent blockage effects on the Ahr River.
3.3 Debris mobilisation
Large woody debris played a critical role in rendering the flood non-linear and difficult to predict, from small headwater channels down to the main streams. Tree logs could have been recruited from forest-covered hillslopes with abundant dead wood due to the drought years of 2018 to 2020 (van der Wiel et al., 2021). However, apart from the linear erosive features described in Sect. 2.2, there was limited field evidence of systematic unconcentrated overland flow on hillslopes, and in no occasion did this point to potential flow depths necessary to entrain logs (Baudrick and Grant, 2000) and route them through a maze of standing trees. The recruitment of large woody debris from riparian zones, where lateral erosion impacted former tree habitats (green line signature in Fig. 3a), is thus much more likely. Aerial imagery collected along main rivers 1 d after the flood (BBK-DLR, 2022) shows substantial removal of trees along the Ahr River and other floodplains. Nevertheless, a quantitative survey of the dead-wood delivery capability of the forested hillslopes and riparian zones still needs to be conducted. Ideally, such a survey would be based on high-resolution point cloud data, for example from dedicated airborne laser scan missions. Understanding the relative importance and pattern of different sources of large woody debris is important not only for restoration efforts in the flood-affected areas but also for mitigating future flood hazards (Lucia et al., 2018). In a preventive manner, especially given the likely increase in extreme events (IPCC, 2021), the general impact of large woody debris on central European landscape dynamics and the susceptibility of different tree species should be investigated.
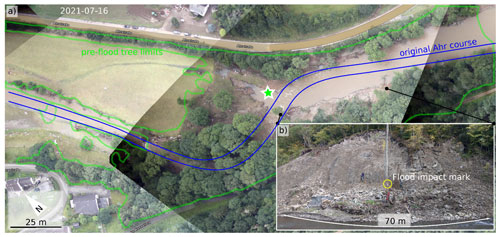
Figure 4Debris mobilisation features in the Ahr valley near Müsch (cf. Fig. 1). Aerial image (BBK-DLR, 2022) taken 1 d after the flood. The light-green outline depicts the tree limit before the flood. Blues lines illustrate the pre-flood course of the Ahr River. (b) View from the green star in panel (a) towards the eroded right bank, which had activated a 16 m high rockslide (persons for scale). Note flood impact mark on a remaining tree at 5 m above current water level. Please note that the date format used in this figure is year-month-day.
The subsequent transport of large woody debris through the river channels had a series of effects (Jochner et al., 2015; Okamoto et al., 2020). In the main streams, clogging of obstacles, mostly bridges, resulted in temporary ponding and backwater effects (Fig. 5a). As a consequence, upstream water levels rose, and the inundated areas grew until the obstacle was either bypassed, overtopped, or destroyed. In the former case, the bypass location experienced increased flow velocity and thus bed shear stress, resulting in focussed erosion. The latter effect has the potential to generate a pulse of water travelling downstream like an outburst flood. Given the presence of many such obstacles along the course of the Ahr valley (62 out of 75 bridges destroyed) and most likely other streams draining the Eifel, the failure of obstacles most likely contributed to non-linear, pulsed hydrograph behaviour, as partly confirmed by affected people and experienced during earlier floods (Roggenkamp and Herget, 2022). However, the large spacing, insufficient time resolution (15 min sampling interval), and eventual failure of existing stream gauges prevent us from resolving this conceptualised hydrograph behaviour and its resulting inundation and shear stress pulses. The traces of these clogs are visible both in the main valleys (BBK-DLR, 2022) and in headwater regions, where we were able to map numerous blockages of first-order streams, either at anthropogenic structures (bridges, water passages, fences) or at narrows formed by riparian trees (Fig. 5b). In many reaches, these blockages were formed at a spacing of a few tens of metres, ponding backwater due to accumulation of organic fine material (Schalko et al., 2018) and implying significant effects already at very small contributing areas. The propagation of non-linear flow effects from small creeks to and throughout the trunk river of a catchment is a crucial step to take for successful future flood impact anticipation.
A further important effect of large woody debris, especially in headwater regions, was the role in ejected coarse inorganic debris from the stream bed (Fig. 4b) onto the floodplain. Also fine material was deposited in front of woody debris obstacles (Fig. 5c) and clogged anthropogenic structures, such as bridges. This readily available fine material is now a temporary source for increased fluvial sediment flux. Hence, even low-intensity floods will be able to carry comparably high concentrations of sediment particles. In contrast, the ejected coarse bed particles are currently removed from the fluvial domain until future bank erosion (or human land use practice) reincorporates them to the channel. Debris also resulted in permanent alterations to stream courses, due to lateral and vertical erosion, and in some steep channel reaches even led to incision into the underlying weathered bedrock as mapped out systematically in headwater reaches. This again resulted in undercutting of the banks and local landslides. The spatial reorganisation of sediment in the fluvial domain as well as overall changes in river geometry and bed properties – from small creeks a few kilometres past the watershed to major rivers like the Ahr – inevitably caused a transient in the catchment reaction to future floods.
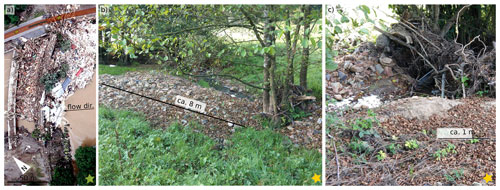
Figure 5Effects of large woody debris. For locations on wider map see stars in Fig. 1. Pair of clogged bridges near Altenahr, bypassed along the left and right bank. Note the bipartition of the collected debris with woody material caught by the downstream road bridge and anthropogenic debris collected later by the upstream railway bridge. Note two remaining standing trees in the river depicting the width of the Ahr River before the flood. Aerial image by BBK-DLR (2022). (b) Huhnenbach near Aremberg about 2 km from its source (see Fig. 1). Note clogging by woody debris at riparian trees and the resulting ejection of coarse bed material out of the channel. (c) Another clogging of the Huhnenbach some 20 m upstream of panel (b), with both ejected coarse debris and deposition of fine sediments in front of the obstacle.
3.4 Anthropogenic dimension
The flood happened in a cultural landscape with a long legacy of human land use. Accordingly, there were typical primary effects of land use, particularly surface features on flood dynamics such as an increased and accelerated surface runoff on cultivated hillslopes (Bronstert et al., 2020), some of which are already mentioned above (e.g. Figs. 2a, 4a; clogged structures, shallow landslides due to undercut, or oversteepened slopes). During our mapping campaign, we observed systematic changes in fluvial erosion features along small headwater channels. Forest-covered floodplains with strong erosional features were connected to virtually unaffected grassland sections, and intact slopes suddenly showed linearly incised sections without a plausible contributing area. In most of these cases, however, we were able to identify artificial subsurface drainage systems, often visible as fragments of drainage pipes. Not surprisingly, according to interviews with residents, systematic tile drainage is a common practice in the area to improve grassland quality or simply to manage the wastewater of dispersed houses. The consequences during the July flood were either an increased discharge contribution where tile drainage remained intact or injection of excess water into the ground where drainage pipes eventually filled up with debris and were clogged. In the former case, the hydrological flashiness of the landscape increased, while in the latter case the result was an elevated susceptibility of hillslopes to failures and incision. Such failures may be local effects, but the increased flashiness had an external effect by increasing the rapid build-up of flood waves in subsequent channels. Since many of the tile drainage and wastewater systems are several decades old and not necessarily documented, including their effects in runoff models will be a challenging though necessary future task.
Both regions, the Ahr valley and Lower Rhine Bay, hold a long legacy of human land use that influence the susceptibility to gravitational mass movements. According to the German landslide database (Damm and Klose, 2015) the pronounced susceptibility to mass movements in the Ahr valley is exemplified by 49 database records from the middle and lower valley sections over about the last 70 years. The records document a preference for fall processes (73 % of the events), in general consisting of rockfalls and boulder falls of low magnitude. Sliding processes amount to 27 %. Inadequate land management as a mass-wasting driver or trigger is conservatively estimated to explain almost 20 % of all cases (Damm and Klose, 2015). In contrast, the Lower Rhine Bay is virtually not susceptible to gravitational mass wasting, except for artificially oversteepened landforms. Over the last 130 years, only 26 events were documented (Damm and Klose, 2015), all of them exclusively located in engineered landscape parts such as slope cuts, hillside fillings, road embankments, waste dumps, open-pit mining, and river management activities. Triggers of the few large-magnitude processes (collectively linked to open-pit mining sites) were predominantly the direct intervention related to mining and the intrusion of external water into the pits, caused by heavy rainfall or flooding. Hence, direct links of flood magnitude to mass-wasting activity are convoluted with land use practice and thus hard to disentangle, at least for past events.
The unprecedented economic damage of more than EUR 30 billion was most likely caused not only by the extent of the affected area (see Sect. 2.1) but also by the velocity of the water flow and the combined impact of water, wood, and debris on buildings and infrastructure. Field inspection revealed inundation depths at buildings of several metres affecting not only cellars and ground floors but also the first floor of many houses (e.g. Roggenkamp and Herget, 2022, and own mapping efforts). Previous major floods in the Ahr valley, Germany, namely 2006 and 2013, also caused inundation damage. However, those previous water levels rose on average to 0.83 and 0.46 m above the ground level, respectively (Thieken et al., 2022). The Ahr valley experienced larger events in the more distant past, such as 1804 (Roggenkamp and Herget, 2022). However, the settlement and land use structure was significantly different from 2021, which makes comparisons of comparably large but temporally more distant events challenging. In addition to inundation depths, high flow velocities and resulting damage due to impacting debris, undermining of paved surfaces and souring of foundations were reported frequently for these valley-confined flood events (Laudan et al., 2017), processes specifically harmful to critical road infrastructure (Kreibich et al., 2002). At downstream reaches, water levels became elevated by deposited debris that reduced accommodation space. None of these processes and damage mechanisms are included in common damage models (Laudan et al., 2017). Likewise, without their inclusion, identification of affected buildings by automatic flood mapping routines (e.g. CEMS, 2022) remains incomplete and requires tedious and time-consuming manual data collection to derive a proper damage estimate. In the Ahr valley, CEMS identified around 5000 affected residential buildings, while an overlay of the reconstructed water mask and an OpenStreetMap data set identified about 7000 buildings. This estimate of 7000 buildings is likely to be too low, as the water masks usually do not map the rapid surface runoff, which could also lead to structural damage of buildings. Hence, the development of concepts and readily applicable tools to reliably estimate the damage particularly to infrastructure is an important emerging goal.
The high death toll along the Ahr River could however not just be related to the shortcomings of damage estimates discussed above. A further effect was the general underestimation of the flood magnitude by locals due to an anticipation legacy. During the 2016 flood in the Ahr valley, discharge was estimated to resemble a 100-year event (Demuth et al., 2022). In 2021, residents tended to recall that past event and the way they coped with it. Since the 2021 event was considerably higher and accompanied by geomorphic processes, this ultimately led to an underestimation of its impact. An online survey that was conducted from August to October 2021 in the affected areas (Thieken et al., 2022) revealed that based on the warnings just 14.8 % of 856 warned residents anticipated massive damage and life-threatening situations (assessed on a Likert scale from 1 to 6). In addition, public authorities, particularly in the district of Ahrweiler, evacuated very late in the evening when the water had already flooded houses. Hence, many residents endured this threatening flood situation on the roofs of their buildings (or drowned).
3.5 Interactions and process connections
Landscape elements and process domains are typically linked by the river network that drains them. Hence, changes in equilibrium processes such as sediment fluxes into a river, ponding, or advancing flood waves can be seen as input signals that are transmitted downstream while becoming modulated in their response. The magnitude and filter function of this modulation can be so strong that the initial input signal is no longer discernible; it gets shredded (Jerolmack and Paola, 2010). One example of this concept, namely the change of the flood's hydrograph by cascades of clogged bridges, has been described in Sect. 2.3. Nevertheless, the modulated response (here the modulated flood wave) still severely impacts downstream reaches – and sometimes even upstream reaches. We follow this concept of signal shredding during landscape interaction through connection mechanisms in the Erft catchment (Fig. 1).
At a comparably small scale, the town of Blessem on the Erft River was subject to such an emerging landscape connectivity case. The excess rainwater of Blessem was collected and channelised in pipes below the main road (Frauenthaler Straße, then passed to Radmacherstraße). These pipes ended in a drainage ditch at the western town limit that routed the water towards the Erft River (Fig. 6b), passing by a gravel pit that was protected by a rampart a few metres high. During the flood, overbank discharge of the Erft River moved water across the main streets of Blessem (light-blue arrows in Fig. 6) and not only injected excess discharge into the drainage pipes but also ran as overland flow along the streets. In addition, Erft overbank discharge was routed over a field west of the town, towards the drainage ditch already carrying the town’s excess water. Whether that excess water caused overspilling of the 2 m deep drainage ditch or if the additional water inflow from the field caused the overspill cannot be resolved here. Regardless, the ditch overtopped and discharge followed the line of steepest descent into the gravel pit, whose protection rampart was not fully closed but had a gap through which the water could enter the pit. As the flow path gradient changed from less than 1 to about 20∘ down the pit slope, the shear stress increased by 2 orders of magnitude (Anderson and Anderson, 2010). This high shear stress, combined with the high erodibility of the underlying material (a thin loess cover on tertiary Rhine gravel), resulted in extremely rapid erosion and the development of an erosional margin (yellow line in Fig. 5) that cut backwards by about 250 m within a few hours. The final shape of the erosional margin shows four discrete tongues of enhanced slope retreat (cf. numbered triangles in Fig. 6), which correspond to the main sources of excess water: the road and drainage pipes running through the town of Blessem (triangle 1); two overflow spots of the Erft River across the field at the edge of the town (triangles 2 and 3); and ultimately, the river Erft itself (triangle 4), which routed its whole discharge into the eroded gravel pit. This led to rapid inundation of the pit, massive deposition of >450 000 m3 of material, enhanced lateral erosion along the (new) outer bank of the diverted river, and significant depth erosion not only of the new course of the Erft River but also in its old bed, forming a knickzone and resulting in a bed elevation lowering of 1.4–1.8 m.
Despite initial media reports of a landslide (i.e. a gravitational mass-wasting process) happening near Blessem, all evidence rather implies that it actually was a process driven by flowing water. That process in turn was the result of a local process connection mechanism: backward erosion of the gravel pit margin. At the same time, the mechanism was also controlled by emerging feedbacks, i.e. the reduction in backward erosion by cessation of overbank discharge through base level lowering of the Erft River as it flowed into the gravel pit. Hence, what happened in Blessem is an example of how geomorphic processes first amplified and later counteracted the impact of a hydrological extreme event.
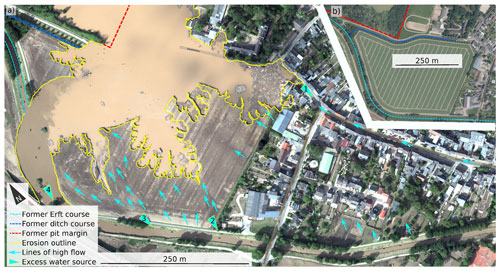
Figure 6Aerial image (BBK-DLR, 2022) of the town of Blessem. (a) Situation shortly after the flood event, with annotated features. The top-right inset (b) shows conditions before the flood. The break in slope along the margin of a gravel pit (red dashed line) had started to erode towards the town by fluvial erosion (yellow line) that formed three individual clusters. The erosion was fuelled by overbank discharge of the Erft River, evading the town of Blessem and moving down the main street, as well as water flowing over the field west of the town margin, following the line of steepest descent. Water flow directions are indicated by blue arrows where visible from aerial imagery. The four numbered blue triangles depict sites of increased water input towards the pit.
At a larger scale, the Blessem site was almost subject to another process connection case, which would have delivered a further significant wave of water. Through the Erft, Swist, and Schießbach rivers, the town is connected to the Steinbach reservoir some 30 km upstream (Fig. 1). The 12 m high earth dam of that reservoir was severely dissected by sustained crown overspill for several hours during the flood event. Gullies of 10 m width and up to 4 m depth formed over a length of about 100 m (quantified by UAV-based – unmanned aerial vehicle – structure-from-motion topographic data). If the Steinbach reservoir dam had failed, another 1.2×106 m3 of water would have moved downstream, most likely refuelling the erosion processes in Blessem with another new flood wave all along the river's course from the reservoir.
A particular phenomenon of the July 2021 flood was the widespread activity of mostly small features that ultimately added up to unexpectedly large effects in the main valleys of the Ahr and Erft rivers. There was not one dominating factor that can explain the event magnitude but rather the interaction of many seemingly unrelated effects, a situation that needs to be considered jointly and conceptualised and implemented in predictive models as well as upcoming mitigation strategies. Some of these isolated effects were straightforward to detect and can be implemented in future strategies, such as insufficiently designed bridges or protective dams. Other effects are inherently difficult to identify and even harder to conceptualise and ultimately implement into models and risk management strategies. Examples of the latter category are tile drainage systems of unknown extent and capacity or injected versus ejected volumes of sediment. These latter effects could, however, be incorporated to the models via Monte Carlo simulations, e.g. considering the full range of potential sediment budget, to at least quantify within the uncertainties bounds of the model estimates.
These emerging effects rendered the flood an extreme beyond the hydrological scope. Hence, this underlines the need for a cross-topic consideration of its internal and external drivers, its effects, and its internal feedbacks. This touches especially on the non-hydrological processes and their representation in posterior models, future predictions, and concepts as well as hazard zone definitions, in addition to the fruitful efforts already emerging from the hydrological realm. For example, while it is evidently important to provide close-range forecasting of potentially inundated areas, it is as important to develop and implement methods to forecast potential effects driven by overland flow and stream discharge. These include outlining potentially unstable hillslopes, riverbed changes, cascading effects, and landscape connectivity effects. Connectivity effects do not necessarily need to be restricted to gravel pits and upstream water reservoirs, as revealed here. More likely are far-reaching effects, for example triggered by blocked tunnels, undermined bridges, valley-damming mass-wasting deposits, and channel straightening that swiftly initiate long-lasting effects.
Upcoming fundamental research needs to quantify the severity and modes of landscape reorganisation as well as constrain the duration of the transient that is now dominating the functional relationships of the affected regions. Key elements of these functional relationships are the reorganisation of the hydraulic geometry, the fluvial and sediment coupling between channels and adjacent hillslopes, and land use and settlement planning. Approaching all these questions crucially depends on dedicated, high-resolution, and continuous empirical data from distributed field instrumentation (also properly operating during future extreme events) in close junction with metrics of societal activities. The 2021 flood clearly demonstrated a flaw in classic flood sensing approaches: collection of just a single metric, water level, by gauges at a few spots along the main stream that overall were destroyed significantly before peak discharge had arrived. To overcome this systematic shortcoming, other systems need to be implemented that are able to collect distributed multivariate data at high temporal resolution and that are not endangered by hostile flood conditions. For example, at scales from global to small catchments, seismic networks from Ekström and Stark (2013), Cook et al. (2021), and Walter et al. (2017) have proven to deliver near-real-time information that allow for the detection, location, and description of catastrophic mass-wasting events. Instead of just the main channel, such high-quality flood-related process information should also be available for headwater regions, where the 2021 flood gained its momentum and non-linearity.
Headwater regions are also the areas where proper flood risk reduction actions can be implemented. The German Federal Water Act allows the federal states to identify flood generating areas which are areas that tend to quickly produce surface runoff. Land management can be regulated in such statutory areas to prevent further deterioration of the infiltration capacities of soils. Currently, only the Free State of Saxony makes use of this option. Besides land management planning and engineering solutions, flood risk reduction decisively needs to consider the sensitisation of citizens to overcome the anticipation bias due to the legacy of experienced events of lower magnitude of less non-linear effects.
All data used in this article are freely available in the references denoted in the text.
MD and UO conceptualised the article and collected field data. MD, RB, KLC, CA, ARB, AL, and AHT contributed to the field survey and mapping efforts. BD contributed the landslide database analysis. FSF, KMN, TS, and AHT performed and evaluated climatic and hydraulic risk and damage analysis. All authors contributed equally to the preparation and writing of the manuscript.
The contact author has declared that neither they nor their co-authors have any competing interests.
Publisher’s note: Copernicus Publications remains neutral with regard to jurisdictional claims in published maps and institutional affiliations.
This research was supported by the HART (Hazard and Risk Team) action of Eifel Flood Event 2021 funded by the Helmholtz Centre Potsdam – GFZ German Research Centre for Geosciences and the DFG (German Research Foundation) Research Training Group NatRiskChange – Natural Hazards and Risks in a Changing World (grant no. GRK 2043/3). Katrin M. Nissen, Felix S. Fauer, and Bodo Damm received funding from the BMBF (German Federal Ministry of Education and Research) project ClimXtreme (grant nos. 01LP1903A/K and 01LP1902H). The publication was supported within the funding programme “Open-Access-Publikationskosten” of the Deutsche Forschungsgemeinschaft (DFG, German Research Foundation; project no. 491075472).
This research has been supported by the Helmholtz-Zentrum Potsdam – Deutsches GeoForschungsZentrum GFZ (HART EifelfloodS), the Deutsche Forschungsgemeinschaft (grant no. GRK 2043/3), and the Bundesministerium für Bildung und Forschung (grant nos. 01LP1903A/K and 01LP1902H).
The article processing charges for this open-access publication were covered by the Helmholtz Centre Potsdam – GFZ German Research Centre for Geosciences.
This paper was edited by Olga Petrucci and reviewed by three anonymous referees.
Anderson, R. and Anderson, S.: Geomorphology: The Mechanics and Chemistry of Landscapes, Cambridge University Press, ISBN-10 0521519780, 2010. a, b
Baudrick, C. and Grant, G.: When do logs move in rivers, Water Resour. Res., 36, 571–583, 2000. a
BBK-DLR: Bundesamt für Bevölkerungsschutz und Katastrophenhilfe, Deutsches Institut für Luft- und Raumfahrt, https://arcgis.bbk.itzbund.de/arcgis/apps/webappviewer/index.html?id=30835aae740e46a59df390eb6192c3bb¢er=6.672,50.903, last access: 11 February 2022. a, b, c, d, e, f, g
Bell, F.: Environmental and engineering Geology, Whittles Pub. Ltd., ISBN-10 1849951241, 2007. a
Bell, R., Dietze, M., Thieken, A., Cook, K., Andermann, C., Beer, A., Vela, A. L., Ries, J. B., Brell, M., Eltner, A., Roessner, S., Schrott, L., Iserloh, T., Seeger, M., and Öztürk, U.: More than just fast flowing water: the landscape impact of the July 2021 west Germany flood, EGU General Assembly 2022, Vienna, Austria, 23–27 May 2022, EGU22-11641, https://doi.org/10.5194/egusphere-egu22-11641, 2022. a
Berger, C., McArdell, B. W., and Schlunegger, F.: Sediment transfer patterns at the Illgraben catchment, Switzerland: Implications for the time scales of debris flow activities, Geomorphology, 125, 421–432, https://doi.org/10.1016/j.geomorph.2010.10.019, 2011. a
Bronstert, A., Crisologo, I., Heistermann, M., Ozturk, U., Vogel, K., and Wendi, D.: Flash-Floods: More Often, More Severe, More Damaging? An Analysis of Hydro-geo-environmental Conditions and Anthropogenic Impacts, in: The Oxford Handbook of Innovation, edited by: Leal Filho, W., Nagy, G., Borga, M., Chávez Muñoz, P., and Magnuszewski, A., 9–12, Springer, Cham, https://doi.org/10.1007/978-3-030-37425-9_12, 2020. a
CDC: Climate Data Centre of the German Weather Bureau, https://www.dwd.de/DE/klimaumwelt/cdc/cdc_node.html, last access: 11 February 2022. a, b, c
CEMS: European Emergeny Management Service, https://emergency.copernicus.eu/, last access: 11 February 2022. a
Cook, K., Rekapalli, R., Dietze, M., Pilz, M., Cesca, S., Purnachandra, R., Srinagesh, D., Paul, H., Metz, M., Dahm, T., Mandal, P., Suresh, G., Cotton, F., Tiwari, V., and Hovius, N.: Detection and potential early warning of catastrophic flow events with regional seismic networks, Science, 374, 87–92, https://doi.org/10.1126/science.abj1227, 2021. a
Damm, B. and Klose, M.: The landslide database for Germany: Closing the gap at national level, Geomorphology, 249, 82–93, 2015. a, b, c
Damm, B., Becht, M., Varga, K., and Heckmann, T.: Relevance of tectonic and structural parameters in Triassic bedrock formations to landslide susceptibility in Quaternary hillslope sediments, Quatern. Int., 222, 143–153, 2010. a
Damm, B., Terhorst, B., and Ottner, F.: Geotechnical properties of periglacial cover beds, in: Mid-Latitude Slope Deposits, edited by: Kleber, A. and Terhorst, B., 153–170, Developments of Sedimentology, ISBN 978-0-444-53118-6, 2013. a
Demuth, N., Gerlach, N., van der Heijden, S., and Johst, M.: Starkregen und Hochwasser in Rheinland-Pfalz im Mai/Juni 2016, http://www.hochwasser-rlp.de/publikationen/bericht_starkregen_hochwasser_juni2016.pdf, last access: 11 February 2022 (in German). a
Dietze, M. and Ozturk, U.: A flood of disaster response challenges, Science, 373, 1317–1318, https://doi.org/10.1126/science.abm0617, 2021. a, b, c
Dietze, M., Cook, K., Illien, L., Rach, O., Puffpaff, S., Stodian, I., and Hovius, N.: Impact of nested moisture cycles on cliff coast failure revealed by multi-seasonal seismic and topographic surveys, J. Geophys. Res.-Earth, 125, e2019JF005487, https://doi.org/10.1029/2019JF005487, 2020. a
DWD: https://www.dwd.de/DE/presse/pressemitteilungen/DE/2021/Downloads/20210825_warnchronologie.pdf? (last access: 11 February 2022), 2021. a
DWD-Agrowetter: https://www.dwd.de/EN/specialusers/agriculture/agriculture_node.html, last access: 11 February 2022. a
Ekström, G. and Stark, C. P.: Simple Scaling of Catastrophic Landslide Dynamics, Science, 339, 1416–1419, https://doi.org/10.1126/science.1232887, 2013. a
Fauer, F. S., Ulrich, J., Jurado, O. E., and Rust, H. W.: Flexible and consistent quantile estimation for intensity–duration–frequency curves, Hydrol. Earth Syst. Sci., 25, 6479–6494, https://doi.org/10.5194/hess-25-6479-2021, 2021. a
Fekete, A. and Sandholz, S.: Here Comes the Flood, but Not Failure? Lessons to Learn after the Heavy Rain and Pluvial Floods in Germany 2021, Water, 13, 3016, https://doi.org/10.3390/w13213016, 2021. a
Giuseppe, F., Simoni, S., Godt, J. W., Lu, N., and Rigon, R.: Geomorphological control on variably saturated hillslope hydrology and slope instability, Water Res. Resour., 52, 4590–4607, https://doi.org/10.1002/2015WR017626, 2021. a
Hu, X., Zhou, L., Liu, W., Wang, H., and Cui, L.: Geomorphic effect of debris-flow sediments on the Min River, Wenchuan Earthquake region, western China, J. Mt. Sci., 18, 2427–2440, https://doi.org/10.1007/s11629-021-6816-1, 2021. a
IPCC: Summary for Policymakers, in: Climate Change 2021: The Physical Science Basis. Contribution of Working Group I to the Sixth Assessment Report of the Intergovernmental Panel on Climate Change, edited by: Masson-Delmotte, V., Zhai, P., Pirani, A., Connors, S. L., Péan, C., Berger, S., Caud, N., Chen, Y., Goldfarb, L., Gomis, M. I., Huang, M., Leitzell, K., Lonnoy, E., Matthews, J. B. R., Maycock, T. K., Waterfield, T., Yelekçi, O., Yu, R., and Zhou, B., Cambridge University Press, Cambridge, United Kingdom and New York, NY, USA, 3−-32, https://doi.org/10.1017/9781009157896.001, 2021. a
Jerolmack, D. and Paola, C.: Shredding of environmental signals by sediment transport, Geophys. Res. Lett., 37, L19401, https://doi.org/10.1029/2010GL044638, 2010. a
Jochner, M., Turowski, J. M., Badoux, A., Stoffel, M., and Rickli, C.: The role of log jams and exceptional flood events in mobilizing coarse particulate organic matter in a steep headwater stream, Earth Surf. Dynam., 3, 311–320, https://doi.org/10.5194/esurf-3-311-2015, 2015. a
Junghänel, T., Bissolli, P., Daßler, J., Fleckenstein, R., Imbery, F., Janssen, W., Kaspar, F., Lengfeld, K., Leppelt, T., Rauthe-Schöch, M., Roeck, M., Walawender, E., and Weigl, E.: Hydro-klimatologische Einordnung der Stark- und Dauerniederschläge in Teilen Deutschlands im Zusammenhang mit dem Tiefdruckgebiet “Bernd” vom 12 bis 19 Juli 2021, Report DWD German Weather Bureau, 2021 (in German). a
Kreibich, H., Thieken, A. H., Grunenberg, H., Ullrich, K., and Sommer, T.: Extent, perception and mitigation of damage due to high groundwater levels in the city of Dresden, Germany, Nat. Hazards Earth Syst. Sci., 9, 1247–1258, https://doi.org/10.5194/nhess-9-1247-2009, 2009. a
Laudan, J., Rözer, V., Sieg, T., Vogel, K., and Thieken, A. H.: Damage assessment in Braunsbach 2016: data collection and analysis for an improved understanding of damaging processes during flash floods, Nat. Hazards Earth Syst. Sci., 17, 2163–2179, https://doi.org/10.5194/nhess-17-2163-2017, 2017. a, b
Lucia, A., Schweintek, M., Eberle, J., and Zarfl, C.: Planform changes and large wood dynamics in two torrents during a severe flash flood in Braunsbach, Germany 2016, Sci. Total Environ., 640–641, 315–326, https://doi.org/10.1016/j.scitotenv.2018.05.186, 2018. a
Molina, A., Govers, G., Van den Putte, A., Poesen, J., and Vanacker, V.: Assessing the reduction of the hydrological connectivity of gully systems through vegetation restoration: field experiments and numerical modelling, Hydrol. Earth Syst. Sci., 13, 1823–1836, https://doi.org/10.5194/hess-13-1823-2009, 2009. a
Okamoto, T., Takebayashi, H., Sanjou, M., Suzuki, R., and Toda, K.: Log jam formation at bridges and the effect on floodplain flow: A flume experiment, J. Flood Risk Manag., 13, e12562, https://doi.org/10.1111/jfr3.12562, 2020. a
Ozturk, U., Wendi, D., Crisologo, I., Riemer, A., Agarwal, A., Vogel, K., López-Tarazón, J., and Korup, O.: Rare flash floods and debris flows in southern Germany, Sci. Total Environ., 626, 941–952, https://doi.org/10.1016/j.scitotenv.2018.01.172, 2018. a
Roggenkamp, T. and Herget, J.: Hochwasser der Ahr im Juli 2021 – Abflussabschätzung und Einordnung, Hydrologische Notizen, 66, 40–49, 2022. a, b, c
Schalko, I., Schmoker, L., Weitbrecht, V., and Boes, R.: Backwater Rise due to Large Wood Accumulations, J. Hydraul. Eng., 144, 04018056, https://doi.org/10.1061/(ASCE)HY.1943-7900.0001501, 2018. a
Schmid-Johannsen, J., Lang, U., and Heiliger, N.: SWR-Datenanalyse zur Flutkatastrophe an der Ahr, SWR Aktuell, 24 November 2021, https://www.swr.de/swraktuell/rheinland-pfalz/flut-in-ahrweiler-so-gross-ist-der-schaden-104.html (last access: 11 February 2022), 2021. a
Schneider, D. and Gebauer, P.: Die Flutkatastrophe im Juli 2021 in Mitteleuropa aus meteorologischer Sicht, Beiträge zur Berliner Wetterkarte, Verein Berliner Wetterkarte e.V. zur Förderung der meteorologischen Wissenschaft, Berlin, Germany, 2021. a, b
Thieken, A. H., Bessel, T., Kienzler, S., Kreibich, H., Müller, M., Pisi, S., and Schröter, K.: The flood of June 2013 in Germany: how much do we know about its impacts?, Nat. Hazards Earth Syst. Sci., 16, 1519–1540, https://doi.org/10.5194/nhess-16-1519-2016, 2016. a
Thieken, A., Kemter, M., Vorogushyn, S., Berghäuser, L., Sieg, T., Natho, S., Mohor, G., Petrow, T., Merz, B., and Bronstert, A.: Extreme Hochwasser bleiben trotz integriertem Risikomanagement eine Herausforderung, https://www.uni-potsdam.de/fileadmin/projects/extrass/Flut2021_StatementThiekenEtAl.pdf (last access: 11 February 2022), 2021. a
Thieken, A. H., Samprogna Mohor, G., Kreibich, H., and Müller, M.: Compound inland flood events: different pathways, different impacts and different coping options, Nat. Hazards Earth Syst. Sci., 22, 165–185, https://doi.org/10.5194/nhess-22-165-2022, 2022. a
Thieken, A. H., Bubeck, P., Heidenreich, A., von Keyserlingk, J., Dillenardt, L., and Otto, A.: Performance of the flood warning system in Germany in July 2021 – insights from affected residents, EGUsphere [preprint], https://doi.org/10.5194/egusphere-2022-244, 2022. a
van der Wiel, K., Lenderink, G., and de Vries, H.: Physical storylines of future European drought events like 2018 based on ensemble climate modelling, Weather and Climate Extremes, 33, 100350, https://doi.org/10.1016/j.wace.2021.100350, 2021. a
Walter, F., Burtin, A., McArdell, B. W., Hovius, N., Weder, B., and Turowski, J. M.: Testing seismic amplitude source location for fast debris-flow detection at Illgraben, Switzerland, Nat. Hazards Earth Syst. Sci., 17, 939–955, https://doi.org/10.5194/nhess-17-939-2017, 2017. a
Weigl, E. and Winterrath, T.: Radargestützte Niederschlagsanalyse und -vorhersage (RADOLAN, RADVOR-OP), promet, 35, 78–86, 2009. a, b
Wenzel, T., Bell, R., Dietze, M., Schrott, L., Beer, A., Braun, A., and Fernandez-Steeger, T.: Hillslope failure due to stream undercutting: The 2021 flood event in the Ahr-valley and resulting mass movements – a multi-method approach, EGU General Assembly 2022, Vienna, Austria, 23–27 May 2022, EGU22-7116, https://doi.org/10.5194/egusphere-egu22-7116, 2022. a