the Creative Commons Attribution 4.0 License.
the Creative Commons Attribution 4.0 License.
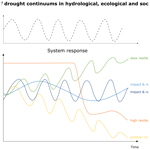
Review article: Drought as a continuum – memory effects in interlinked hydrological, ecological, and social systems
Sarra Kchouk
Alessia Matanó
Faranak Tootoonchi
Camila Alvarez-Garreton
Khalid E. A. Hassaballah
Minchao Wu
Marthe L. K. Wens
Anastasiya Shyrokaya
Elena Ridolfi
Riccardo Biella
Viorica Nagavciuc
Marlies H. Barendrecht
Ana Bastos
Louise Cavalcante
Franciska T. de Vries
Margaret Garcia
Johanna Mård
Ileen N. Streefkerk
Claudia Teutschbein
Roshanak Tootoonchi
Ruben Weesie
Valentin Aich
Juan P. Boisier
Giuliano Di Baldassarre
Yiheng Du
Mauricio Galleguillos
René Garreaud
Monica Ionita
Sina Khatami
Johanna K. L. Koehler
Charles H. Luce
Shreedhar Maskey
Heidi D. Mendoza
Moses N. Mwangi
Ilias G. Pechlivanidis
Germano G. Ribeiro Neto
Tirthankar Roy
Robert Stefanski
Patricia Trambauer
Elizabeth A. Koebele
Giulia Vico
Micha Werner
Droughts are often long-lasting phenomena, without a distinct start or end and with impacts cascading across sectors and systems, creating long-term legacies. Nevertheless, our current perceptions and management of droughts and their impacts are often event-based, which can limit the effective assessment of drought risks and reduction of drought impacts. Here, we advocate for changing this perspective and viewing drought as a hydrological–ecological–social continuum. We take a systems theory perspective and focus on how “memory” causes feedback and interactions between parts of the interconnected systems at different timescales. We first discuss the characteristics of the drought continuum with a focus on the hydrological, ecological, and social systems separately, and then we study the system of systems. Our analysis is based on a review of the literature and a study of five cases: Chile, the Colorado River basin in the USA, northeast Brazil, Kenya, and the Rhine River basin in northwest Europe. We find that the memories of past dry and wet periods, carried by both bio-physical (e.g. groundwater, vegetation) and social systems (e.g. people, governance), influence how future drought risk manifests. We identify four archetypes of drought dynamics: impact and recovery, slow resilience building, gradual collapse, and high resilience–big shock. The interactions between the hydrological, ecological, and social systems result in systems shifting between these types, which plays out differently in the five case studies. We call for more research on drought preconditions and recovery in different systems, on dynamics cascading between systems and triggering system changes, and on dynamic vulnerability and maladaptation. Additionally, we advocate for more continuous monitoring of drought hazards and impacts, modelling tools that better incorporate memories and adaptation responses, and management strategies that increase societal and institutional memory. This will help us to better deal with the complex hydrological–ecological–social drought continuum and identify effective pathways to adaptation and mitigation.
- Article
(2516 KB) - Full-text XML
-
Supplement
(931 KB) - BibTeX
- EndNote
Drought is a creeping phenomenon (Wilhite and Glantz, 1985) with unclear definitions of when a dry spell develops into a drought (Hall and Leng, 2019; Kiem et al., 2016; Slette et al., 2019). This is what we read in the introduction of almost every drought paper and what we have been taught at school or university. However, in drought monitoring, analysis, forecasting, and management, drought is still framed as an event. For example, most disaster databases record only within-year events and do so in a binary way (drought/no drought; e.g. EM-DAT, 2023). However, multiple failed rainy seasons cause exponentially more harm than a single failed season, as for example recently seen in the Horn of Africa (Amha et al., 2023). Also in ecosystems, consecutive droughts cause legacies, affecting these ecosystems' long-term resilience (Müller and Bahn, 2022). In many places, such as the Netherlands, drought monitors and management committees are only operational in the summer period when most impacts are expected or are only put in place once a defined drought event is underway (KMNI, 2023). This is problematic because drought impacts in a single season or year are strongly dependent on what happened in previous seasons and years (i.e. antecedent conditions and baseline vulnerability to drought) and on what happens afterwards (i.e. responses to and recovery from drought). This is what we call “memory”; a process that is prevalent both in the hydrological system, ecosystem, and social system and in their interactions. For example, the responses of a hydrological system to alterations of dry and wet periods are related to the memory of previous conditions. Here, we argue that the event-based approach to understanding and managing drought needs to change if we want to better mitigate drought impacts on both ecosystems and society. We make this argument by exploring and discussing memory effects in interacting hydrological, ecological, and social systems based on the scientific literature and narratives from five global cases.
Drought has different faces, and complexities are inherent in each aspect of drought, including hazard, impacts, and overall risk. Drought hazards can manifest in different parts of the hydrological system, propagating from meteorological drought to soil moisture drought and hydrological drought, with different spatial and temporal characteristics (Van Loon, 2015). There are different temporal dimensions to drought, which range from flash droughts to mega-droughts (Christian et al., 2021; Cook et al., 2022; Ionita et al., 2021; Pendergrass et al., 2020). Flash droughts are driven by a short but extreme precipitation deficit (often co-occurring with high evapotranspiration rates (Shah et al., 2022; Sungmin and Park, 2024) and mega-droughts by a less extreme, but very prolonged, reduction in precipitation and/or increase in evaporative demand (Ault et al., 2016). Not only drought development but also recovery from drought varies in space and time and between different parts of the hydrological system. This means that the end of a drought is not always clear and that dry conditions may linger for a long time (Parry et al., 2016; Tijdeman et al., 2022). Drought hazards are quantified with a range of different indices (such as the standardised precipitation index (SPI) – McKee et al., 1993; the standardised precipitation evapotranspiration index (SPEI) – Vicente-Serrano et al., 2010; or the self-calibrated Palmer drought severity index – Wells et al., 2004), many of which allow for considering different levels of severity and different time periods (accumulation of precipitation over several timescales). However, in event-based analyses, these are again reduced to a single timescale and a threshold is used to define a distinct start and end date for a specific drought event (Brunner et al., 2021; Kchouk et al., 2022; Van Loon, 2015).
Drought impacts on ecosystems and society are wide-ranging and extend across a variety of temporal and spatial scales. The delineation of what a drought impact is and when it starts and ends is not straightforward (Hall and Leng, 2019; Slette et al., 2019), and the timescales of drought impacts are highly variable (de Brito et al., 2020). Drought impacts are often gradual in time, for example an increased walking distance to collect water due to the drying up of boreholes, progressive vegetation stress due to decreasing soil moisture levels, reduced energy production or goods transported due to reduced river water levels, or affected livelihoods leading to school dropouts. Some of these changes are even reported not as impact but rather as a way of coping with drought. This makes relating drought impacts to drought hazard indicators challenging (Bachmair et al., 2016; Shyrokaya et al., 2024). A way to include social dynamics is to add vulnerability factors (Blauhut et al., 2016). Other studies use continuous impact data and relate these to gradual drought severity levels with continuous indices (e.g. crop yield; Madadgar et al., 2017). This approach can capture non-linear relationships but still does not consider the fact that vulnerability can also change during drought, thereby affecting future drought impacts (i.e. vulnerability is dynamic; de Ruiter and van Loon, 2022).
Drought risks are complex (Blauhut, 2020). Interactions between hazard, vulnerability, and impacts are not always one-way, and feedback, human (re)actions, and cascading effects make the relationship between drought hazard and impacts non-linear and dynamic (de Brito, 2021; Kchouk et al., 2022; Wens et al., 2019). For example, when agricultural crops are affected by soil moisture drought, impacts may initially be mitigated by applying irrigation (e.g. from groundwater), but later this water abstraction enhances hydrological drought, which in turn impacts other sectors dependent on groundwater (e.g. drinking water supply; Pauloo et al., 2020). These interactions and non-linearities are often not included in drought hazard impact studies (Wens et al., 2019), and studies on future drought risk only consider changes in drought hazard, keeping exposure and vulnerability fixed in time (Hagenlocher et al., 2019). In addition, the perception of drought severity, impacts, planning, and management can also considerably differ among different societies or communities, as well as over time, and may not always align with the actual observed drought severity and impacts (Teutschbein et al., 2023).
An event-based approach to defining droughts historically has been developed for several reasons. Firstly, it was important to distinguish drought as an extreme event from natural variability. For extreme-value statistics and other quantitative analyses, drought event characteristics were needed based on a clear delineation of drought vs. no-drought (Hisdal et al., 2000). Several studies, however, have shown that the selection of a timescale for the analysis has a strong influence on the results, e.g. for the relation between heatwaves and societal response and impacts (De Polt et al., 2023), ecological impacts of drought (Gouveia et al., 2017), and linking drought indicators to societal impacts (Bachmair et al., 2015). Secondly, it can be helpful to frame drought as disaster for political reasons or to release funding based on drought declarations and triggers (Botterill and Hayes, 2012; Estrela and Sancho, 2016; Monte et al., 2020). A disaster risk framing of drought requires a hazard event to be distinguished with a specific exposure and vulnerability related to the hazard event (Blauhut, 2020). There have been calls for moving from crisis management to more risk-based management of drought (Estrela and Sancho, 2016; Sivakumar et al., 2014; Wilhite, 2017; Wilhite et al., 2000), and this suggestion is already being implemented in some national or regional drought management plans. Guidelines and declarations like the guidelines for drought management plans (Iglesias et al., 2009), the 2013 High-level Meeting on National Drought Policy (HMNDP) declaration (Sivakumar et al., 2014), the National Drought Management Policy Guidelines (Wilhite and Pulwarty, 2014), and the Handbook of Drought Indicators and Indices (Svoboda and Fuchs, 2016) have been instrumental in advancing drought management. Regional examples from central and eastern Europe (Fatulová, 2014; GWP CEE, 2014) and the Horn of Africa (GWP, 2015) highlight the development of drought management, supporting capacity building and increased resilience to drought impacts. International collaborative efforts like the WMO–GWP Integrated Drought Management Programme (IDMP) have been very important for highlighting and sharing these efforts. However, research has shown that drought management plans are mostly strong in emergency management and less developed on determining long-term goals and implementing measures to achieve these (Fu et al., 2013) and that they could improve on including vulnerability and impacts (Urquijo-Reguera et al., 2022). Additionally, in some countries, the drought disaster framing has been found to be unhelpful in drought management. For example, Australia removed droughts from the natural disaster policies in 1989 because droughts started to be viewed as a continuing risk related to climate variability and drought declarations triggering government support caused debate and inequalities (Botterill and Hayes, 2012). Here, we argue that, taking an event-based view of drought, i.e. monitoring or assessing drought as a snapshot in time (Bastos et al., 2023), analysing each drought driver or impact separately, and focusing on emergency management and drought emergency declarations, oversimplifies the complexity of drought and its dynamics (Hagenlocher et al., 2023). Such an event-based approach overlooks important periods that shape antecedent conditions and recovery from drought, as well as the cascading and compounding effects of drought processes, dynamic vulnerability, and feedback between hazard and impact. Moreover, because of the complex interactions between drought and multiple systems, we support the notion that understanding drought requires considering not only physical (hydro-meteorological) processes but also ecological (environmental) and social (economical, political) processes to assess drought risks. Concurrently, scholars have recently argued that drought risk should be seen as systemic, i.e. resulting from complex interdependencies and feedbacks between compounding and concurring hazards, as well as possible tipping points (Hagenlocher et al., 2023; Sillmann et al., 2022). To address this issue, a systems theory perspective is needed. Therefore, in this paper, we combine the frameworks of social–ecological systems and earth system science and apply these to the temporal dimensions of drought. We first explain the theoretical frameworks used (Sect. 2); then we discuss memory in hydrological, ecological, and social systems (Sect. 3); and next we compare the emerging properties and feedbacks within and between these systems (Sect. 4). We base our analysis on a review of the scientific literature and on five case studies in different parts of the world (see Supplement Sects. S1–S5). We conclude with an outlook providing recommendations for further research and improved monitoring and management (Sect. 5). Consideration of these aspects is needed for a new perspective on drought, wherein we conceptualise drought as a continuum and consisting of interacting hydrological–ecological–social memory processes.
In this paper we build on the concepts of complex systems and systems thinking to conceptualise drought as a hydrological–ecological–social system and to draw on elements from social–ecological systems, socio-hydrology, and earth system science. Our specific focus is the dynamic aspects of these systems interacting over time as they are affected by and create system memory. In this section, we first introduce key overarching concepts relevant to our conceptualisation of the drought system and its temporal dimensions.
The field of systems thinking defines complex systems as composed of a set of elements (which can be systems themselves) that have connections between each other (Jackson, 2019; Shaked and Schechter, 2017). The interactions between these interconnected elements can lead to unexpected emergent results (Westra and Zscheischler, 2023). Elements can interact and feed back at different scales, creating a multidimensional complex adaptive system (Rammel et al., 2007). Systems theory is applied to, for example, agriculture, natural resource management (Ison et al., 1997), and disaster recovery (Bahmani and Zhang, 2021).
Social–ecological systems (SESs) are examples of complex adaptive systems characterised by integrated bio-physical and socio-cultural processes (Ahmed and Abdalla, 2005; Delgado-Serrano et al., 2015; Ostrom, 2009; Tellman et al., 2018). Socio-hydrology or hydrosocial systems can be seen as a specific type of SES revolving around the interactions between people and water (Konar et al., 2019; Sivapalan et al., 2012; Wesselink et al., 2017). Many studies, for example, use socio-hydrology to understand and model the complex dynamics of flood risk resulting from the interplay between floods and people (Di Baldassarre et al., 2013; Vanelli et al., 2022). Earth system science (ESS) focuses on the complex adaptive components of the earth system and their interactions (Steffen et al., 2020). ESS is strongly based in the natural sciences (meteorology, climate physics, environmental science) but has more recently recognised the important role of humans as agents of change of the earth system (Alessa and Chapin, 2008). One difference between SESs and ESS is the scale at which they are studied, with ESS focusing on the planetary scale (Steffen et al., 2020).
Table 1Examples of drought as a continuum in hydrological systems, ecosystems, and social systems based on specific studies.
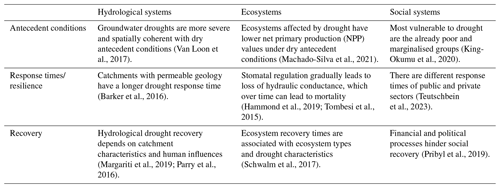
Within complex social–ecological or earth systems, the interactions between the elements or subsystems happen across both spatial and temporal scales (Konar et al., 2019; Vanelli et al., 2022). In this paper, we are interested in temporal aspects. Naylor et al. (2020) state that to understand complex systems and their emergent properties, it is necessary to examine the changes in relationships between system elements over time. The concept of time is studied extensively in the separate systems – the hydrological system (Koutsoyiannis, 2013), ecosystem (Jackson et al., 2021), and social system (Peixoto and Rosvall, 2017) – despite common features between them. Aspects like antecedent conditions, response times to disturbances, and recovery to the original state (or transition to a new state) jointly shape the response of a system to external drivers. These factors determine whether the system changes quickly or slowly, depending on the system's memory. The memory of a system refers to its ability to retain information about past states, conditions, and experiences, which influences its current behaviour and response to future events. Memory is often manifested through legacies and responses. Legacies are the lasting effects of past conditions that might continue to influence the system's structure, function, and behaviour over time. Responses are the manifestation of how quickly the system reacts to disturbances and adapts to changes. A system with a long memory retains past influences for a longer period, leading to slower responses and longer legacies, while a system with a short memory quickly responds to disturbance and has short legacies (Gunderson and Holling, 2002; Kchouk et al., 2023; Redman and Kinzig, 2003).
The memory of the systems within a complex system strongly determines the emerging properties, such as (i) self-organisation and emergence, (ii) non-linear behaviour and tipping points, (iii) state shifts and feedback loops, and (iv) resilience and adaptation (Carmichael and Hadžikadić, 2019; Preiser et al., 2018). Such properties are particularly evident when examining the co-evolution of human and water systems across time. For example, Srinivasan et al. (2012) introduced the concept of “syndromes” to conceptualise and describe the evolving nature of human–water interactions over time. These syndromes represent specific patterns of water use, reflecting the dynamic state of the system as it changes and adapts with time. Similarly, Roobavannan et al. (2017) modelled a “pendulum swing” in the management of the Murrumbidgee Basin in Australia, which is in fact a shift from agricultural to environmental water allocation. This shift reflects the “memory properties” of systems as it was shaped by accumulated experiences, past policies, and societal values, showing how historical experiences influence current practices.
Time is an important element in the development of drought and drought impacts, as recognised by previous studies (Hall and Leng, 2019; Tijdeman et al., 2022; Wilhite and Glantz, 1985; WMO, 2021), and time characteristics have been studied empirically in the separate systems (see some examples in Table 1). In the next sections, we explore and discuss the concept of memory shaping drought over time from different perspectives: hydrology, ecology, and social science. Next, we analyse potential temporal interactions across these systems to understand how they impact the broader drought system across time.
3.1 Hydrological system
The emphasis on drought as a hydrological extreme event has led to drought detection and definition using indices specified over defined timescales (McKee et al., 1993; Mishra and Singh, 2010) or considering a limited range of lagged hydro-meteorological variables (Mishra and Singh, 2011). However, it is increasingly recognised that hydrological droughts result from complex interactions between multiple bio-physical processes and human influences (Van Loon et al., 2016). This implies that hydrological droughts occur not as singular events but rather as a result of the continuous evolution of multiple hydrological fluxes and states. Therefore, we cannot fully characterise droughts without considering the (wet and dry) hydrological conditions that either precede or follow what is considered a drought event, as well as how baseline conditions may be shifting over time due to climate change. The duration for which these hydrological conditions need to be considered to understand the evolution of drought and subsequent recovery primarily depends on the processes that contribute to catchment memory (Stoelzle et al., 2020).
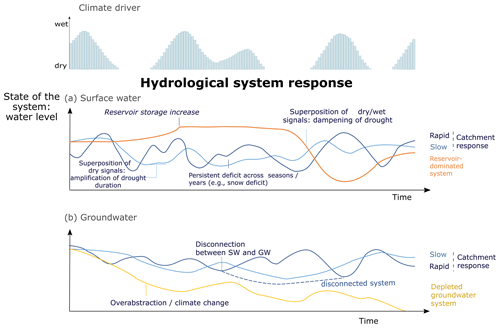
Figure 1Drought in surface water (SW; a) and groundwater (GW; b) in different catchments in response to a climate driver (e.g. precipitation, recharge), with a fast or slow catchment response (dark- and light-blue lines), with a reservoir (orange line), and with groundwater depletion (yellow line). The “drought wave” decreases in amplitude but increases in wavelength as the catchment storage increases. This results in a superposition of anomaly signals in the hydrological system. When groundwater becomes disconnected from surface water, memory increases and response to precipitation is strongly reduced.
Catchment memory, in the context of drought, modulates the cumulative effects of anomalous meteorological and hydrological conditions and their persistence over time and thus the severity, duration, and recovery of droughts (Alvarez-Garreton et al., 2021). This memory depends on the heterogeneous and spatially distributed characteristics of the catchment, such as topography, land cover, soil types, storage properties, and variability in hydro-climatic conditions (Cranko Page et al., 2023; Fowler et al., 2020; De Lavenne et al., 2022). For instance, catchment memory in surface-water-dominated catchments may be quite short, depending on soil moisture and vegetation memory (Ghajarnia et al., 2020; Gu et al., 2023; Fig. 1a, dark-blue line). By contrast, in groundwater-dominated catchments, catchment memory may typically be longer, as groundwater acts as a storage reservoir that buffers short-lived rainfall anomalies and sustains baseflow in rivers and streams (Sutanto and Van Lanen, 2022). Such a long memory will, however, lead to slower recovery, particularly if groundwater levels have been significantly depleted due to more persistent rainfall deficits (Fig. 1b, light-blue line). This was found during the 2018–2022 drought in groundwater-dominated systems in the eastern part of the Netherlands, which showed minimal or no recovery despite the drought being interspersed with relatively wet conditions in the winter of 2019–2020 (Brakkee et al., 2022; see the Rhine River basin case study, Sect. S5). High meteorological variability can dissipate catchment memory given rapid and frequent changes in hydrological states, especially in systems with shallow groundwater tables where excess water cannot be stored and the system is reset during wet periods (Appels et al., 2017; van der Velde et al., 2009). However, large subsurface storage with deep groundwater levels tends to attenuate the effects of variability in precipitation and evapotranspiration on the hydrological system. This then contributes to the accumulation of drought deficits and the lagging and pooling of meteorological drought events, thus extending the recovery process (Sutanto and Van Lanen, 2022). Other forms of storage can also contribute to long catchment memory, such as extensive wetlands and lakes (Gu et al., 2023). Furthermore, human-made storage reservoirs can increase catchment memory and buffer drought (Ribeiro Neto et al., 2022), though only up to certain critical thresholds such as when the reservoir falls empty (Rangecroft et al., 2019; Fig. 1a, orange line). Recovery in such reservoir-influenced catchments may be slower (Margariti et al., 2019; see also the northeast Brazil case study, Sect. S3).
Catchment memory varies across different climate types. In arid and semi-arid climates, propagation from meteorological to hydrological drought may be slower than in wet–tropical climates (Gevaert et al., 2018; Odongo et al., 2023). This may be exacerbated by land–atmosphere interactions, which can lead to the self-propagation of droughts, thus extending them in space or time (Miralles et al., 2019; Schumacher et al., 2022). Catchment memory also varies in climates with distinct seasonality, such as tropical savannas, snow-dominated catchments, or Mediterranean-type climates (Gevaert et al., 2018; Seager et al., 2019) where drought propagation has a strong intra-seasonal (De Lavenne et al., 2022) or even multiannual timescale (Gevaert et al., 2018). For example, in the Andes Cordillera, snow deficits lead to streamflow deficits not only during the summer melting season but also in the following autumn season (Alvarez-Garreton et al., 2021; see the Chile case study, Sect. S1). Similarly, winter snow droughts in the snow-dominated catchments of the Alps affect summer discharges of the river Rhine (Ionita and Nagavciuc, 2020; Khanal et al., 2019), while in the winter of 2022–2023, unprecedented dry and warmer-than-normal conditions over the Italian Alps caused critical hydrological conditions in the Po and Adige rivers in the ensuing spring (Colombo et al., 2023). Another example is the Mediterranean region, where precipitation is highly seasonal due to winter storms. The weakening of the storm systems combined with long–dry summers leads to a precipitation deficit and, thus, increased drought risk in the region (Cook et al., 2014; Ionita and Nagavciuc, 2021).
Catchment memory can, therefore, connect climate and hydrological anomalies across different temporal scales. Precipitation anomalies occurring at a particular time of the year can be compounded and lead to long-memory streamflow anomalies later in the year (Mudelsee, 2007). Figure 1 schematically shows how the superposition of different drought signals and hydrological states with long and short memory may result in either amplifying or dampening the duration and severity of hydrological droughts. However, the interaction of these signals is not always linear, as witnessed by the unexpectedly quick recovery in groundwater systems in Germany (Tijdeman et al., 2022: see the Rhine River basin case study, Sect. S5).
It is worth noting that the processes that constitute catchment memory are not stationary. Unprecedented climatic conditions such as multi-year droughts may alter how a catchment responds to precipitation and/or how surface and groundwater systems interact (Fuchs et al., 2019; Fig. 1b, dashed line). This can lead to persistent shifts in rainfall–runoff relationships (Eltahir and Yeh, 1999; Kleine et al., 2021) and less runoff than expected (Alvarez-Garreton et al., 2021; Fowler et al., 2020; Saft et al., 2015). Further, catchments may not always fully recover and return to their original states after protracted droughts end, leading to new persistently low-flow states due to changes in the dominant hydrological processes and catchment memory (Peterson et al., 2021; Fig. 1b, yellow line).
In addition to the effects of protracted dry conditions, climate change can also lead to non-stationary catchment responses through aridification as a result of greater atmospheric water demand, increased evaporation, and lower soil moisture (Boisier et al., 2018; Overpeck and Udall, 2020), as experienced in the Colorado River basin in the southwestern USA (Colorado case study, Sect. S2). Climate warming may lead to a modified drought response by shifting the hydrological regime of a basin from snow-dominated to rainfall-dominated. A shift from snow to rain in winter may reduce catchment memory, dampening winter droughts and amplifying spring and summer droughts, as observed in Sweden (Arheimer and Lindström, 2015; Teutschbein et al., 2022) and the western USA (Siirila-Woodburn et al., 2021). Climate change also affects snow and glacier dynamics and storage and therefore the drought buffering effect these aspects have (van Tiel et al., 2023).
Finally, human activities can change catchment memory processes, such as through the overexploitation of groundwater leading to depletion and degradation of aquifer systems. Such perturbations modify drought propagation mechanisms and may increase drought impacts by reducing water security, potentially leading to permanent loss of natural water storage (Fig. 1, yellow line; Alvarez-Garreton et al., 2024; Chile case study, Sect. S1), and by causing land subsidence due to compaction (e.g. San Joaquin and Central Valley in California; Ojha et al., 2018; Smith et al., 2017). Land use change can also result in changes in catchment memory. For example, large-scale tree restoration or afforestation can result in either more or less water availability, depending on the balance between increased evapotranspiration and increased precipitation (Galleguillos et al., 2021; Hoek van Dijke et al., 2022). Similarly, the effect of urbanisation on streamflow drought is a balance between decreased water storage due to increased imperviousness and increased water storage because of increased sewage return flows and pipe leakage (Van Loon et al., 2022).
3.2 Ecosystem
Drought has widespread impacts on terrestrial ecosystems globally and is a major driver of variability in the global carbon cycle (Ray et al., 2015; Reichstein et al., 2013; Schwalm et al., 2017; Stocker et al., 2019). The impacts of droughts on ecosystems depend on drought characteristics, ecosystem memory, and the interactions between ecosystems and their environment and are not necessarily detrimental (Cranko Page et al., 2023; Kannenberg et al., 2020; De Long et al., 2019; de Vries et al., 2023; Wu et al., 2022). For example, plants in many semi-arid and arid systems have developed drought-tolerant traits, such as deep roots, thick and leathery leaves, drought deciduousness, and high fire resistance, allowing them to cope with drought and cascading impacts such as wildfires (Blumenthal et al., 2020; Jacobsen et al., 2008). Moreover, droughts can promote development and retention of biodiversity (e.g. in drought- and fire-dependent ecosystems) (Agee, 1996). Drought can also stabilise the ecological landscape and reinforce community resilience (Lloret et al., 2012; Fig. 2, orange line). However, shifts in drought patterns, e.g. due to anthropogenic climate change, compounded with other human pressures on ecosystems, can alter ecosystem composition and functioning, in turn affecting ecosystem resilience and potentially leading to critical memory effects (Bastos et al., 2023; Crausbay et al., 2020; Kannenberg et al., 2020).
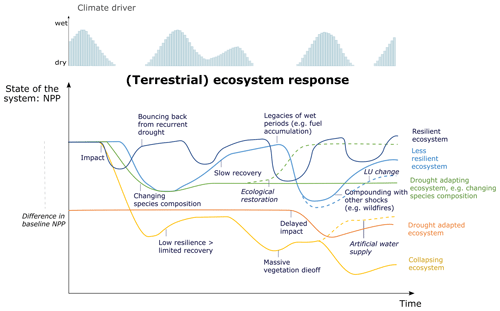
Figure 2Drought in a variety of different ecosystems in response to a climate driver (e.g. precipitation, recharge), with drought-adapted ecosystems (orange line) starting at a lower baseline, drought-adapting ecosystems (green line) changing in response to drought, more or less resilient ecosystems (dark- and light-blue lines) impacted by and recovering from drought, and collapsing ecosystems (yellow line) gradually decreasing due to recurrent droughts. NPP denotes net primary production, and LU denotes land use. The dashed lines represent ecosystem management and other human influences.
The impacts of drought on ecosystems take place at multiple timescales, from very short (hours to days) to very long (years to decades; Fig. 2, dark-blue and light-blue lines). The effects of drought on ecosystems can vary depending on vegetation type (Ruehr et al., 2019), soil type (Buttler et al., 2019), historical and concurrent climate (Ruiz-Pérez and Vico, 2020; de Vries et al., 2023; Zipper et al., 2016), microclimate (Suarez and Kitzberger, 2008), preconditions (Bastos et al., 2020) and the timing of drought within growing seasons (Hahn et al., 2021; Iizumi et al., 2018) due to distinct phenological sensitivity to climatic conditions (Wu et al., 2021). The initial impacts are on plant physiological processes (Hsiao, 1973; Li et al., 2023), as low water availability reduces turgor pressure in leaf cells, stomatal conductance, and xylem conductivity and generally results in decreases in whole-plant hydraulic conductance affecting water and carbon exchanges through the soil–plant–atmosphere continuum (McDowell et al., 2022; Tyree and Ewers, 1991). These effects cascade to affect overall plant primary productivity (Griffin-Nolan et al., 2018), growth (Kannenberg et al., 2019), and carbon allocation (Hartmann et al., 2020), as well as plant–plant (Zhang et al., 2019) and plant–insect (Kolb et al., 2016; Öhrn et al., 2021; Raderschall et al., 2021) interactions, or even cause plant death.
Drought can furthermore negatively affect soil carbon (C) storage by reducing below-ground plant C inputs and altering their quality (Fuchslueger et al., 2016; de Vries et al., 2019; Williams and de Vries, 2020), reducing microbial activity and decomposition of soil organic matter and affecting plant and microbial communities and their interactions (Schimel, 2018). Experiments in grasslands have shown rapid responses of plant and microbial growth and community composition to drought but slow responses of total soil C pools (Aanderud et al., 2015; Placella et al., 2012; de Vries et al., 2016). Twenty years of chronic summer drought has caused persistent shifts in soil fungal and bacterial communities and has reduced microbial biomass and soil C under grasses but not under heather plants (Gliesch et al., 2024). This highlights the role of plant community composition and drought characteristics in affecting soil C pool dynamics at different temporal scales (Fig. 2, blue line).
While some ecosystems seem to recover quickly after a single dry period, others take 2 or more years to recover (Anderegg et al., 2015; Schwalm et al., 2017; Wu et al., 2022). Vegetation sensitivity to drought has been reported to increase in the season following an initial dry period in some ecosystems (Bastos et al., 2021; Machado-Silva et al., 2021; Nagavciuc et al., 2023; Wu et al., 2022). These memory effects can be caused by several mechanisms. First, plant activity recovers in several hours to years, depending on water stress characteristics (e.g. intensity and duration), the vulnerability of the plant tissues, and memory in the soil–plant–atmosphere system (e.g. from previous periods). Photosynthetic processes generally recover quickly (e.g. within hours or days), but hydraulic damage or failure requires a longer time, provided recovery is possible at all (Adams et al., 2017; Choat et al., 2018; Ruehr et al., 2019). The amount of precipitation after the drought period also plays a role. For example, in the Yangtze River basin (China), grasslands had already recovered with 50 % of normal precipitation, while forests required at least near-normal precipitation to fully recover (Huang et al., 2021). Second, drought reduces plant uptake of soil nutrients, leaving a larger nutrient pool available for post-drought plant growth than that available in normal conditions. This could lead to fast-growing plants proliferating and could decrease the ecosystem's ability to cope with a second drought (de Vries et al., 2018). Third, in natural ecosystems, the cascading short- or medium-term effects of droughts can increase background mortality (McDowell et al., 2022) and shift plant functioning and drought strategies, with long-term altered species composition and sensitivity to climate (Crausbay et al., 2020; Griffin-Nolan et al., 2019; Fig. 2, green line). Drought also shifts the composition of soil microbiota, including the balance of mutualists and pathogens, potentially leaving plants more vulnerable to subsequent drought events (de Vries et al., 2023). Fourth, chronic drought can cause reduced soil carbon inputs, a loss of soil carbon, and changes in soil physical properties (Zhang et al., 2018), reducing the soil water-holding capacity and rendering ecosystems more vulnerable to subsequent drought. These post-drought memory effects propagate through the whole ecosystem, represented as changes in ecosystem functioning beyond the current growing season (e.g. defoliation detected by aerial surveys in Meddens et al., 2012, and large-scale satellite-sensed vegetation greenness by Wu et al., 2022), losses in woody biomass for years ahead (Anderegg et al., 2015), and post-drought tree mortality or major die-off in the low-resilience ecosystems (Allen et al., 2010, 2015; Fig. 2, yellow line). A case in point is the recent drought in central Europe, where strong legacy effects during a multi-year drought caused massive vegetation die-off (see the Rhine River basin case study, Sect. S5). At the ecosystem scale, divergent impacts and recovery responses in more diverse systems might result in weaker drought legacy effects compared to less diverse and more vulnerable systems (Yu et al., 2022). Some of these mechanisms also play a role in ecosystems dominated by annual plants, such as agroecosystems, altering vulnerability to subsequent droughts (Renwick et al., 2021), but no legacy effects of drought on crop production have appeared at a national scale (Lesk et al., 2016).
In managed ecosystems, like agroecosystems and managed forests, the effects of droughts are compounded with management strategies and land use practices. For example, the vulnerability to water stress is reduced by irrigation (Luan and Vico, 2021; Zipper et al., 2016) (Fig. 2, dashed yellow line). It might also be reduced by species diversification in space (e.g. species-rich grasslands, mixed-species forests, intercropping, and drought-resistant species; Grossiord et al., 2020; Haberstroh and Werner, 2022; McCarthy et al., 2021; Sears et al., 2021; Wright et al., 2021) or time (i.e. crop rotations; Bowles et al., 2020; Marini et al., 2020; Renwick et al., 2021) but exacerbated by forest clear-cutting through land–atmosphere interactions (Pongratz et al., 2009; Wu et al., 2017; Fig. 2, dashed light-blue line). Soil management, e.g. tillage, affects soil properties and functioning and hence the response to drought in ways depending on local conditions (Pittelkow et al., 2015; Schneider et al., 2017). The timescales for the implementation of mitigating actions (e.g. irrigation) or for the effects to emerge (e.g. crop rotations) can be long (Marini et al., 2020; Renwick et al., 2021). Most of the cascading effects that appear in natural ecosystems are similar in managed ecosystems, but these can be buffered or amplified by specific management practices (e.g. rotation periods, age structure, stand density, diversification). Nevertheless, in managed ecosystems, plant species composition is defined by management itself and not by plant community evolution, albeit still in the context of existing climate and risk avoidance preferences. Over time, increasing droughts could, for example, promote the adoption of climate-resilient crops and varieties (Acevedo et al., 2020).
The prolonged effects of drought could also increase the frequency of severe wildfires (see the Chile and Colorado River basin case studies, Sects. S1 and S2), but actual impacts on ecosystems may be delayed by years or decades depending on fire management strategies. While wildfire consequences can manifest in individual dry years (Abatzoglou and Williams, 2016; Holden et al., 2018; Littell et al., 2016), tree damage and mortality driven by the effects of earlier extended droughts increase the fire severity, fire frequency, and burned area in historically fire-adapted forests (Stephens et al., 2018). However, wet periods are also important for wildfire risk. For example, some of the current wildfire crises in the western USA stem, in part, from a relatively wet period in the 1950s and 1960s that facilitated fire suppression in forests with historically frequent fires and was followed by deepening droughts and extended dry spells (Abatzoglou and Williams, 2016; Holden et al., 2018) in forests with subsequently more abundant fuels (Hessburg and Agee, 2003). In addition to fire-induced tree mortality, tree mortality can also occur after drought because of the increased risk of insect outbreaks due to the lower resistance of drought-stressed trees (Fettig et al., 2019; Kolb et al., 2016; Luce et al., 2016; McDowell et al., 2022; Fig. 2, yellow line). As a consequence of these long-term and cascading impacts, some ecosystems are now seeing extensive ecological transformation (Crausbay et al., 2020; Steel et al., 2023).
Some of these processes feed back into the development of hydrological drought. For example, with increasing water limitation, some plants are able to increase their water use efficiency, therefore buffering water loss through transpiration (Flach et al., 2018; Peters et al., 2018) and maintaining photosynthesis, although contrasting patterns have been found globally (Yang et al., 2016). Differences in water use and drought stress responses underlying different vegetation types can, therefore, contribute to asymmetries in the development of soil moisture anomalies during drought (Bastos et al., 2020; Flach et al., 2018). Other factors, such as the earlier onset of the phenological cycle, may further contribute to exacerbating summer drought (Lian et al., 2020).
3.3 Social system
Less water than normal in part of the hydrological system can have a significant negative impact on social systems, including on livelihoods, food security, and health, but is only impactful when it exceeds the capacity to manage the water deficit, for example when it affects an already vulnerable population (Raju et al., 2022). Similarly to ecosystems, the impacts of droughts on social systems depend on the severity of the water deficit and the capacity of the system to cope with and adapt to such conditions. Social impacts of drought arise when policies, regulations, and other drought management actions fail or are inadequate and society cannot cope with dry conditions. Additionally, different groups or sectors of society can be impacted by the same drought but in different ways and at different times (Stahl et al., 2016; Wlostowski et al., 2022); they may also respond differently (Teutschbein et al., 2023). For instance, rainfed agriculture is sensitive to meteorological and soil moisture drought, while hydropower and inland navigation are sensitive to hydrological droughts (Van Loon, 2015; Teutschbein et al., 2023). This mirrors how different ecosystems and vegetation types respond variably to drought based on their specific characteristics and resilience (see Sect. 3.2). Consequently, the timing of drought impacts in each context is related to the speed of drought propagation through the hydrological cycle (Van Lanen et al., 2016) (Fig. 1) and societal processes (such as water allocation laws, priority rules) that can cause amplified, attenuated, or lagged effects (Fig. 3a, dark-blue and light-blue lines). This can be observed when immediate drought impacts are delayed by prevention and mitigation measures (e.g. irrigation) but will be felt later, and potentially more severely, when the drought propagates to surface water or groundwater (van Dijk et al., 2013). Drought impacts on society can also be reduced with emergency measures like food aid (Fig. 3a, dashed line), which may not be sustainable in the long term if drought persists. In some sectors, societal responses can even increase the impacts of drought, such as when drought not only reduces water availability but also increases water demand, the combination of which stresses public water supplies (Di Baldassarre et al., 2018).
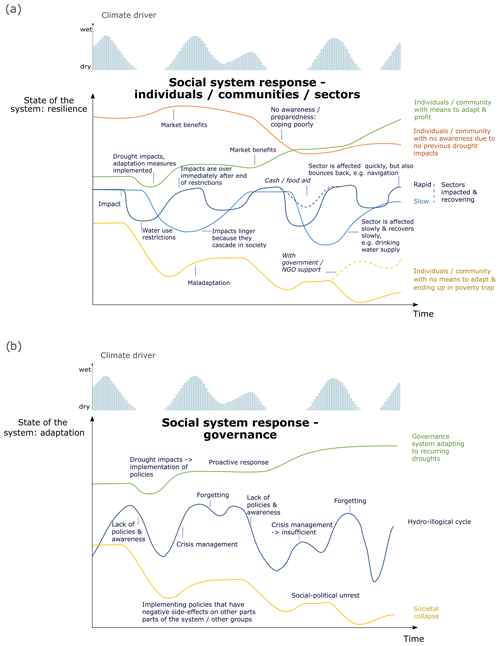
Figure 3Drought in the social system in response to a climate driver (e.g. precipitation, recharge): (a) individuals, communities, and sectors and (b) governance. (a) Different communities have different baseline resilience (starting point on y axis) and different means to adapt and respond (green and yellow lines), different sectors can be impacted and recover on different timescales (dark- and light-blue lines), and communities can be highly resilient but also unaware and therefore more affected when drought hits (orange line). (b) Governance systems can follow the hydro-illogical cycle (Wilhite, 2011) of emergency response and forgetting (blue line), implement policies that allow for proactive response and preparedness (green line), or create a maladaptive system that could end in societal collapse (yellow line).
Similarly to their propagation through the hydrological cycle (Sect. 3.1) and akin to the way drought affects various physiological and community-level plant processes over time (Sect. 3.2), drought impacts cascade through society and the economy with different speeds. They affect different groups and regions with different intensities and timings and potentially far from where the drought originated (de Brito, 2021). Drought impacts are often gradual changes in factors that can also be influenced by other processes (e.g. decline in crop yield, energy production losses, reductions in goods transported via rivers), which makes it difficult to define whether anomalies are impacts of a drought or caused by something else, as well as when they start and end (Hall and Leng, 2019). Moreover, some of these impacts can be the result of the drought itself (lower water availability) or be related to responses to drought (lower water allocation). In response to drought, individuals can take a variety of measures to mitigate impacts such as leaving some portion of agricultural land fallow, minimising transport loads, or decreasing outdoor or inessential water use. Decision-makers may decide to implement water use restrictions (van Oel et al., 2018; Ribeiro Neto et al., 2022) or restrictions on navigation or cooling water discharge in order to preserve limited water supplies for more critical uses. Migration can also be seen as either a coping mechanism or an adaptive measure against drought (Falco et al., 2019; Vinke et al., 2020). Migration can also increase the vulnerability of the migrating group (e.g. decreased health/financial resources) or put extra stress on the water resources of the receiving area, potentially also affecting the original communities there. These drought-related decisions and restrictions also impact society, as they shift exposure to water deficits from one group or system at risk to another.
Drought impacts may also linger long after the drought hazard has ended (WMO, 2021), creating indirect impacts such as disrupted international trade (Carse, 2017); temporary or permanent unemployment; business interruption (Ding et al., 2011); loss of income (Zaveri et al., 2023); mental health issues (Vins et al., 2015); diseases due to poor water and air quality (Charnley et al., 2021; Mora et al., 2022); and food insecurity, malnutrition, starvation, and widespread famine (Bailey, 2013; UNDDR, 2021). However, drought impacts may also be positive for some groups; for example, increased crop prices may result in higher incomes for those farmers who do not suffer a significant production loss (Ding et al., 2011) (Fig. 3a, green line), and dry and warm weather may boost tourism, especially in cold, wet climates, e.g. mountain areas (Koutroulis et al., 2018; Liu, 2016; Wlostowski et al., 2022).
How society is impacted by and responds to drought is not dependent on only a single drought, however. It is also shaped by a complex interplay of dry and wet cycles, socio-political preconditions, socio-economic dynamics, adaptive behaviour and memory at multiple governance levels, and other processes directly or indirectly related to drought. In this section, we discuss three points that illustrate how drought functions as a continuum in the social system.
Firstly, social vulnerability to drought is dynamic, both within and between droughts (de Ruiter and van Loon, 2022). Dynamic vulnerability means that communities' susceptibility to drought impacts can change over time, influenced by varying levels of exposure, sensitivity, adaptive capacity, and collective memory, as well as underlying inequalities (with regard to water access and who benefits from the top-down reactive drought management) (IPCC, 2014; UNDDR, 2021). Critically these aspects of vulnerability are also influenced by evolving drought conditions and past droughts, among other, more external, factors. For instance, extended dry situations may gradually erode communities' financial resources (Enqvist et al., 2022; Kchouk et al., 2023; Savelli et al., 2021) (see the northeast Brazil case study, Sect. S3), physical health (Belesova et al., 2019; Sena et al., 2017; Treibich et al., 2022) and mental health (O'Brien et al., 2014), access to education (Hyland and Russ, 2019), family and community harmony (Dean and Stain, 2007), and more (Keshavarz et al., 2013), in ways that exacerbate ongoing or future vulnerability (Fig. 3a, yellow line). For instance, repeated drought can deplete a household's resources, making migration or other coping and mitigation choices impossible and trapping societies in a vicious cycle of increasing vulnerability (Black et al., 2011; Black and Collyer, 2014; Nawrotzki and DeWaard, 2018).
How a community recovers after a drought event also influences future vulnerability. Societies that recover quickly after a drought have been found to be less vulnerable to the next drought compared to societies that recover slowly (Di Baldassarre et al., 2018; Kchouk et al., 2023; Weiss and Bradley, 2001). This is similar to ecological systems where the speed of recovery from drought impacts influences future resilience and vulnerability. However, returning quickly to a past state without considering the need to build resilience to future events can exacerbate vulnerability and undermine long-term resilience (Koebele et al., 2020). Indeed, successive droughts, or droughts compounded by other hazards, extend the recovery time of affected communities. Therefore, vulnerability is not static, and a fixed level of vulnerability cannot be defined for a specific event. Using pre-drought estimates of vulnerability and averages calculated over extended periods can underestimate the compounding nature of vulnerability.
There is also a strong imprint of long-term social, political, and economic processes unrelated to drought on social vulnerability and therefore on drought risk. For example, in the 2018 Cape Town drought (often referred to as “Day Zero”), Apartheid-era social processes influenced vulnerability to drought through historical, spatial, and economic segregation, which led to long-term unequal access to water (Enqvist and Ziervogel, 2019; Savelli et al., 2021) and made some communities inherently more vulnerable (Fig. 3a, different baselines). An aggressive water-metering campaign by the government, coupled with massive increases in the price of water, further strained these communities' already-limited financial resources (Enqvist et al., 2022).
Secondly, adaptation happens in response to past, ongoing, and/or expected drought experiences, which influences future drought risk (Kreibich et al., 2022). While short-term coping measures, such as buying food or water, are stopped when they are not needed anymore, long-term adaptation measures, like implementing irrigation or changing livelihood, have a long legacy. Adaptation happens on the scale of individuals and communities, as well as governments, and is strongly related to individual and collective memory. While drought events may leave a significant impact on people's memory due to the immediate and tangible effects experienced (van Duinen et al., 2015; Gebrehiwot and van der Veen, 2021; Griffiths and Tooth, 2021; Taylor et al., 1988), this memory likely fades over time, especially if something else eventful such as flooding happens (Garcia et al., 2022). Recency bias in human memory (related to the availability heuristic; Garcia et al., 2022; Tversky and Kahneman, 1973) gives greater importance to the most recent events. This can lead to a gradual decrease in the perceived risk of droughts and the neglect of long-term drought management practices (Fig. 3a, orange line).
Communities can retain the memory of previous droughts through institutional arrangements (Howden et al., 2014), cultural practices, and collective experiences (Pandey and Bhandari, 2009; Salite and Poskitt, 2019; Shiferaw et al., 2014). In drought-prone areas of sub-Saharan Africa, for example, farmers have adopted different drought-risk coping strategies to reduce their risk of drought (see the Kenya case study, Sect. S4). These include choosing specific crop varieties, temporal adjustments of the cropping calendar, changes in weeding and fertilisation practices, and use of soil and water conservation practices. Some of these strategies, which originated as coping mechanisms, have become an integral part of the farming system and have reduced overall risk (Pandey and Bhandari, 2009; Shiferaw et al., 2014; Fig. 3a, green line).
Droughts trigger not only individual and community action, but also management response from governments across different levels. Droughts can drive short- and long-term policies and decision-making, similarly to how management strategies and land use practices in managed ecosystems can mitigate or exacerbate the effects of drought (see Sect. 3.2 and Figs. 2 and 3a, yellow lines). Short-term crisis management is most common, including emergency relief, such as water trucks and cash transfers, targeted at specific areas and affected groups (Barendrecht et al., 2024; Wilhite, 2017). However, governments often deal with each drought as a “new” or unique event, possibly because of a low memory of “creeping disasters” like drought (Ulibarri and Scott, 2019; Wilhite and Glantz, 1985) (illustrated in the hydro-illogical cycle; Fig. 3b, blue line). This makes it less likely that long-term proactive measures are implemented for drought compared to other natural hazards (Hurlbert and Gupta, 2016). For example, in the Netherlands, severe drought events (1976, 2003) resulted in fewer structural measures than flood events (1953, 1993) (Bartholomeus et al., 2023). In South Africa, Vogel and Olivier (2019) analysed responses to droughts over time and found “reaction but little effective `deep' thinking about drought. The persistent truths of recurring drought, a failure to learn from the process of drought rather than the event, the problems of the scientific uncertainty linked to droughts and the usual crisis response to drought made by a select few, are all shown to be threats to ensuring adaptation to repeated droughts in the future” (Vogel and Olivier, 2019).
Furthermore, applying emergency measures to address the persistent impacts of droughts may conceal the need for long-term management strategies and lead to unintended consequences in other systems, such as in the case of Chile, where the law has suspended the obligation to maintain ecological flows in several basins for over 8 consecutive years as a means to mitigate socio-economic drought impacts (see the Chile case study, Sect. S1; Alvarez-Garreton et al., 2023). Long-term management starts to emerge after multiple drought events as a lagged-effect scenario. For example, in Chile and Argentina after 4 years of drought emergency, drought was no longer seen as a “hazard” but as the normal state (Hurlbert and Gupta, 2016). According to Nohrstedt (2022) “transformation does not materialize as an immediate response to dramatic agenda-setting disaster, but will rather emerge gradually through time due to accumulated experience from multiple events” (Nohrstedt, 2022, p. 432; Fig. 3b, green line). A review by Mendoza et al. (2024) found that in several studies, communities were able to distil insights from previous drought experiences through farmer field schools and other collaborative learning spaces. Engaging in long-term collaborative learning enabled communities to see which adaptive strategies worked best in specific conditions. However, management or adaptation not only is implemented in response to drought, but also can be implemented in anticipation of (increasing) drought risk. Recently, climate change projections, with special attention paid to the risks of droughts, have been actively integrated into policy development and decision-making processes in Europe. This is evidenced by the initiatives and strategies outlined in the first European Climate Risk Assessment (EUCRA, 2024) and the European Drought Risk Atlas (Rossi et al., 2023) through advanced modelling, systematic risk assessments, and addressing interconnected and cross-border impacts.
Thirdly, responses to drought can later turn out to be maladaptive. Maladaptation (or rebounding vulnerability) occurs when the outcome of adaptation measures ends up increasing the vulnerability of a community over time (Juhola et al., 2016; Schipper, 2020) (Fig. 3b, yellow line). For example, increasing water storage and supply with reservoirs provides a buffer during dry periods but can also lead to a form of the safe development paradox called the “reservoir effect” (Di Baldassarre et al., 2018). Over-reliance on reservoirs can increase social exposure and vulnerability when a drought occurs. Short-term adaptation measures can also erode the conditions for sustainable development by consuming the adaptive capacity of a community and preventing it from taking measures with long-term benefits. For example, if during a previous drought, there had been an increase in groundwater pumping that continued after the drought, the impacts of a second drought may be experienced more quickly due to the added effect of groundwater pumping (Pauloo et al., 2020). Moreover, changes in rainfall patterns can affect water user behaviour, which again may influence the sustainability of small-scale rural water service providers due to high intra-seasonal revenue variability (Armstrong et al., 2022).
Maladaptation impacts vary across society as a result of social processes including poverty, inequality, power asymmetries, and ineffective decision-making. For example, in Cape Town during the 2018 drought, the wealthiest populations, who already had the highest consumption rate prior to the crisis (Enqvist and Ziervogel, 2019), could also afford to implement coping strategies such as drilling private groundwater wells (Simpson et al., 2019), which ultimately decreased their vulnerability to drought compared to the pre-crisis level but lowered water availability for those who could not afford to drill deeper wells. In rural areas of Chile, people rely on self-organised communities with inadequate infrastructure for providing subsistence drinking water, leading to water cuts that were remedied by cistern trucks. Cistern trucks have become a non-structural reactive measure to address permanent water access requirements in rural areas. On the other hand, people in urban areas rely on water sanitation companies and have not been affected by water cuts because these companies have adequate infrastructure (see the Chile case study, Sect. S1).
4.1 Similar emergent temporal patterns between the systems
In the hydrological, ecological, and social systems studied in Sect. 3, common patterns are visible, corresponding to the systems theory element of “self-organisation and emergence” (Sect. 2). We see that the dynamics of drought in all systems can be characterised as a combination of different fluctuations, cycles, gradual changes, and shocks that emerge from memories and responses within the specific system, following the systems theory element of “resilience and adaptation” (Sect. 2). This leads us to define a typology of the drought continuum, with four archetypical temporal drought trajectories (Fig. 4):
-
Impact and recovery. The system is affected by drought but subsequently bounces back. Depending on the type of system, this impact and recovery can happen quickly or slowly, related to short or long memory (type 1a and 1b, Fig. 4). Superposition of signals with different time frames can occur, like we discussed for the hydrological system (Sect. 3.1).
-
Slow resilience building. The system adapts well to drought, and drought resilience increases over time. We see this for example in selected social systems (e.g. drought-resilient farming systems; Sect. 3.3) and in ecosystems (e.g. drought-tolerant traits; Sect. 3.2).
-
Gradual collapse. The system becomes more vulnerable with each drought and changes to a negative state. In the ecosystem, we see this as a result of long-term legacy effects and compounding processes (Sect. 3.2). In the social system, this happens as a combination of high baseline vulnerability and maladaptation (Sect. 3.3).
-
High resilience–big shock. The system has a baseline of high resilience and is not affected initially, but it is impacted more after prolonged or successive droughts that lead to critical transitions. We see this most clearly in the social system, where an initially high resilience leads to a lack of awareness and preparedness until a severe drought causes a tipping point (Sect. 3.3). However, also in ecosystems, dry conditions can trigger a catastrophic shift in seemingly stable systems (Scheffer et al., 2001).
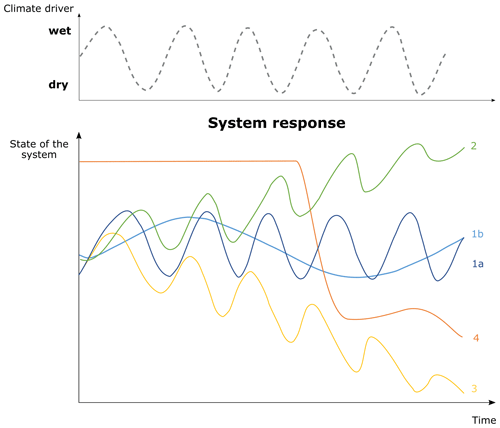
Figure 4Typology of drought continuums emerging from the analysis of temporal drought trajectories in the hydrological, ecological, and social systems: (1) impact and recovery (1a, a quickly responding system; 1b, a slowly responding system), (2) slow resilience building, (3) gradual collapse, and (4) high resilience–big shock.
4.2 Interactions leading to critical transitions overflowing between systems
Because the systems are intrinsically intertwined, a change in one system leads to a change in another system. This can trigger a system to change trajectory, leading to type transitions. These interactions correspond to the systems theory elements of “non-linear behaviour and tipping points” and “state shifts and feedback loops” (Sect. 2).
For example, a high-resilience–big-shock social system may be using water resources unsustainably and depleting groundwater, shifting the hydrological system from an impact-and-recovery system to a gradual-collapse system (point 1, Fig. 5a). At first, this may bring benefits to society and not impact the ecosystem too much, but at a certain moment a tipping point is reached where the ecosystem also moves into gradual collapse, for example when groundwater-dependent ecosystems dry out completely and are lost (point 2, Fig. 5a).
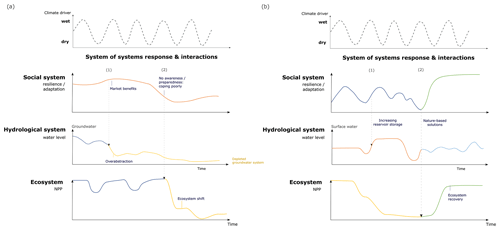
Figure 5Example pathways of connected systems moving between types: (a) from an impact-and-recovery system to a gradual collapse system and (b) from an impact-and-recovery system to a slow resilience building system.
On the other hand, an impact-and-recovery social system that implements reservoirs can create a high-resilience–big-shock hydrological system but at the same time can cause a gradual collapse in the ecosystem (point 1, Fig. 5b). If society then shifts to a slow-resilience-building social system, such as through the adoption of nature-based solutions, this may nudge the hydrological system into impact and recovery, and then the ecosystem can also evolve into slow resilience building (point 2, Fig. 5b).
4.3 Case studies of hydrological–ecological–social drought continuums
We explored different drought typologies, system interactions, and type transitions in five case studies (Chile, Colorado River basin, northeast Brazil, Kenya, Rhine River basin; Sects. S1–S5).
4.3.1 System types
System types are apparent in the case studies. For example, in Kenya (Sect. S4), we see the impact-and-recovery typology. Short-duration, heavy rainfall has been demonstrated to play an important role in groundwater recharging after drought, resulting in the recovery of the hydrological system (Matanó et al., 2024). The case in northeast Brazil (Sect. S3) shows signs of a gradual-collapse typology due to maladaptation. In some communities, the introduction of a reservoir aimed at reducing drought vulnerability ultimately proved to be maladaptive. As the reliance on external labour for income shifted to intensive irrigated agriculture following the reservoir's implementation, the community's financial resources progressively eroded due to recurring droughts (Kchouk et al., 2023). In the Rhine River basin (Sect. S5), we find an example of the high-resilience–big-shock type. The system is highly managed and engineered, vulnerability is low, and there is a lack of awareness, which means that drought impacts are often not felt by society. Impacts are compensated for due to market price effects, are passed on in time, or are passed down to other systems. Drought management ignored connections between systems and longer-term impacts until this was no longer possible in the recent droughts in 2018–2020 (Bartholomeus et al., 2023).
4.3.2 Systems influencing each other
We also see examples of one drought system influencing another. The multi-year drought in Chile (Sect. S1) instigated changes in the hydrological system, which were influenced by the social system. During the drought, snow-dominated basins in the Andes generated on average 30 % less streamflow than previous single-year droughts due to long catchment memories (impact and recovery – type 1b). In some rural areas, this memory effect leading to lower flows has overlapped with water extractions for human activities in downstream sections (impact and recovery – type 1a), leading to an amplification of drought signals in lowlands that has resulted in the drying out of lakes and pumping wells supplying water for human consumption (impact and recovery – superposition of signals due to interaction between hydrological and social systems). Also, the drought response of ecosystems has been strongly influenced by both the hydrological and the social systems. Before the drought, wildfires were concentrated between November and April, but now they extend from October to May, increasing the occurrence period from 6 to 8 months (impact and recovery – change of type 1a to type 1b due to interaction between hydrological systems and ecosystems). This increase is related to the drier conditions during the multi-year drought, but it is also modulated by local anthropogenic perturbations. More than 70 % of the megafires (>50 000 ha of burned area) of the last 4 decades have occurred during drought, where 50 % of the burned area corresponds to monocultures of exotic species (mainly pine and eucalypt trees). It is also worth noting that 99 % of wildfires in Chile are caused by human actions, whether they are accidental or intentional. In these examples, the system maintains its typology, and the interactions cause a lengthening or enhancement of the drought memory, leading to changes in response and recovery.
This was also apparent in the Colorado River basin (Sect. S2), which has been in a long drought for over 2 decades (2000 to the present). Despite being punctuated by periods of high winter precipitation, the basin has experienced more very dry years than normal and major reservoirs have been drawn down to record low levels. Here, the hydrological system strongly influences the ecosystem. Extended drought, coupled with aridification, threatens the health of various riparian environments and endemic species, some of which already face the risk of extinction (gradual collapse – speeding up due to interactions between hydrological systems and ecosystems). It may also lead non-native species to dominate over native species (Rogosch et al., 2019). The hydrological system has also influenced the social system, especially governance. In the early years of the drought, policymakers took incremental actions because they did not proactively estimate or understand how severe the drought would become. This has led to the need for repeated policy changes, which challenges policymaker and stakeholder capacity, as well as public support (Deslatte et al., 2023; Garcia et al., 2020) (impact and recovery – type 1a). The occasional “wet” year may also have undermined drought adaptation as decision-makers “forget” or “ignore” the drought (impact and recovery – less memory due to interaction between hydrological and social systems), though these years may also provide helpful buffers to make adaptation measures successful (e.g. adding a small amount of water to storage reservoirs). The highly polycentric governance system of the basin – meaning that many different actors and sectors have the authority to make decisions about water management – may also challenge drought governance due to actors' different values, legal rights, and experiences of drought.
4.3.3 Feedback between systems
Feedback between systems results in a two-way influence on memory. The high concentration of small dams in northeast Brazil (that have been built to protect against drought; Sect. S3) affect the memory of the watershed. During multiannual droughts, these dams remain dry longer due to low precipitation and high evaporation rates. This in turn reduces hydrological connectivity by decreasing runoff and recharge to the large reservoirs. These reservoirs are vital for urban water supply, and the delay in recharge prolongs the impacts of the drought (Ribeiro Neto et al., 2022). So this is an example of how the hydrological system changes from impact and recovery type 1a to impact and recovery type 1b due to the interaction between social and hydrological systems and of how the social system then also changes from impact and recovery type 1a to impact and recovery type 1b due to the interaction between hydrological and social systems.
Feedback between two systems can also result in (potential) type transitions, for example the social system causing a gradual collapse of the hydrological system, which then triggers a high-resilience–big-shock mode in the social system. In Chile (Sect. S1), a large portion of the drinking water supply in the capital is obtained from long-memory groundwater systems that are consistently being depleted and that may already have been disconnected from subsurface flows and recharge (e.g. 300 m deep pumping wells were recently inaugurated as a key drought adaptation strategy). This generates a false perception of not being under drought because, despite a decade-long drought and depleted surface reservoirs, people are not experiencing water shortages in Santiago. This may lead to increased vulnerability – the opposite aim of the adaptation strategy – by over-relying on a water supply system that is based on an invisible reservoir that has an unknown but finite volume which is not being replenished (Alvarez-Garreton et al., 2024). A similar situation emerged in the Colorado River basin (Sect. S2), where, due to high levels of natural interannual variability, major reservoirs were constructed in the previous century to store water from snowmelt for use in dry years (i.e. “buying time” during drought years). However, this response may be maladaptive in the future as the climate changes (more dry years, higher temperatures, more precipitation as rain rather than snow), leading to other unsustainable actions that forebode gradual collapse (i.e. increase groundwater pumping when surface water is not available) (Garcia et al., 2020). This has already led to significant water supply challenges, especially in the lower half of the basin. However, sustained collaboration among policy actors on water sustainability may push the social system towards a mode of slow resilience building (Karambelkar and Gerlak, 2020; Koebele et al., 2020).
4.3.4 Dynamic shift of the hydrological–ecological–social system
Interactions between all three subsystems can result in a dynamic shift of the hydrological–ecological–social system. In Kenya (Sect. S4), the more frequent droughts in the 21st century pose new challenges for the social, ecological, and hydrological systems. Key social processes affecting land use, most prominently agricultural expansion, have affected hydrological and ecological systems. Land use changes have affected how meteorological drought propagates to hydrological drought and have led to a weakening of ecological buffers to drought, such as riparian forests (impact and recovery – change of type 1b to type 1a due to interaction between hydrological systems and ecosystems). While policy responses to drought in Kenya have historically been reactive, there has been an emergence and expansion of public dams, water reservoirs, and irrigation systems for crop farming in previously pastoral areas. While being able to buffer droughts (impact and recovery – change of type 1a to type 1b due to interaction between hydrological and social systems), new socio-hydrological dynamics are triggered, such as reservoir effects (high resilience–big shock), divergent paths of vulnerability among water infrastructure users, and pressure on surrounding natural resources (gradual collapse). Continually adapting to the new drought reality, a young generation of pastoralists are starting to support more strict grazing zone management, which may reduce degradation of vital hydrological and ecological buffers to drought (slow resilience building). This is an example of where shifts in the social system can trigger type transitions so that the combined hydrological–ecological–social drought system can move to a slow-resilience-building typology.
Similarly in the Rhine River basin (Sect. S5), historically, (ground)water levels and vegetation interact dynamically and have been able to recover from shocks (impact and recovery). Over time, artificial drainage and over-abstraction have resulted in depleted groundwater, and agricultural management and pollution have rendered ecosystems highly vulnerable. The combination of these factors has led to a stressed system that was strongly impacted in the 2018–2020 drought and showed very limited recovery (gradual collapse due to the effect of social systems on hydrosystems and ecosystems). However, this recent event also sparked interest and awareness, which resulted in improvements in drought monitoring and forecasting, the development of new policies, and the implementation of more sustainable adaptation (nature-based solutions). For example, in the Netherlands, regional water authorities can implement surface water use restrictions during drought, but since the multi-year drought, there has been an increased awareness that groundwater use should also be restricted, with the aim to prevent long-term effects on the hydrological system and potential cascading effects on the social system and ecosystem (Bartholomeus et al., 2023). These are the first signs that the gradual collapse is being changed to slow resilience building, in which changes in the social system are improving the hydrological system and the ecosystem and finally benefiting the entire hydrological–ecological–social system.
The use of systems theory to explore the temporal dimensions of the hydrological–ecological–social drought continuum has provided important insights. These insights could be used in future studies and practices to improve drought management. Here, we discuss some suggestions.
5.1 Scientific outlook
Research on drought as a continuum should encompass both enhanced process understanding and improved tools and methods. We suggest the following:
-
Hydrological modelling tools used for drought analyses should better represent memories in the hydrological system. These memories were found to contribute to a better forecast skill for streamflow drought (Du et al., 2023; Sutanto et al., 2020; Sutanto and Van Lanen, 2022), but not all hydrological memories are currently represented well in modelling tools, especially multiannual dynamics (Fowler et al., 2020) and catchment memory processes related to snow, groundwater (Tallaksen and Stahl, 2014), and vegetation (Troch et al., 2013). Further, analyses and predictions should incorporate non-stationarity in hydrological–ecological–social processes and future extremes (Brunner et al., 2021; Samuel et al., 2023). Substantial improvement can come from better incorporating these dynamics.
-
Modelling of ecosystem dynamics and memory should also be further developed. State-of-the-art process-based ecosystem models already mostly include soil water dynamics and some of their delayed effects on physiological processes but should also consider longer-term key legacy effects of droughts and other disturbances via, for example, the explicit consideration of groundwater dynamics (Mu et al., 2021), drought-induced structural damage (defoliation, xylem damage), and mortality and hence ecosystem composition or enhanced vulnerability to pests (Kolb et al., 2016) and wildfire (Hantson et al., 2016; Luce et al., 2016). Similarly, soil-mediated long-term legacy effects, e.g. via microbial community composition and activity, and soil carbon effects on soil hydraulics or interactions with nutrient availability are generally neglected. Typically, these modelling limitations arise from the currently limited mechanistic understanding of these processes, especially at regional to global scales.
-
Analyses of the social processes underlying drought risk should better include temporal dynamics and effects of social memory for individuals, communities, and governance systems. Given the role of socio-economics, inequalities, perceptions, and other social processes in defining drought risk and recovery, the ability of governance systems to maintain institutional memory and effectively manage this integrated system is particularly relevant. Additionally, scholars should investigate how polycentric systems, which are often praised as a solution for complex water management, may actually produce maladaptive outcomes in the presence of poor coordination, power asymmetries, a lack of leadership, disincentives for proactive change, and more (Biddle and Baehler, 2019; Lubell et al., 2014; Morrison et al., 2019). Empirical research on these processes would give important insights.
-
Research needs to be developed to better understand the role of drought preconditions and post-drought recovery in different systems. These would need to take into account dynamic vulnerability (de Ruiter and van Loon, 2022) and the interaction between long-term changes and short-term dynamics in different components of the systems. Long-term changes can include climate change, ecosystem composition changes, socio-economic changes, and changes in land and water use or management, which all influence catchment, ecosystem, and social memories. From the analysis of these short- and long-term dynamics, the occurrence of types and type transitions can be inferred. It would be informative to investigate under what conditions these types and type transitions occur. For this, we suggest analysing contrasting cases in different parts of the world by combining observational data with modelling.
-
More research is needed on interactions and feedback between systems related to drought impacts and responses (Vanelli et al., 2022). For example, groundwater depletion should be analysed using approaches that include the complexity of hydrological–social interactions over time (Schipanski et al., 2023). Also studies on maladaptation to drought should consider the interactions and feedback within the hydrological–ecological–social system (Adla et al., 2023). Furthermore, we advocate for more collaboration between climate scientists and ecologists (Mahecha et al., 2022).
-
Tools for analysing drought as a continuum need to better accommodate interactions between systems and shifts in types. This could be done, for example, by combining the analysis of historical causal pathways (Srinivasan et al., 2012) with the development of future adaptation pathways (Haasnoot et al., 2013). A promising approach is that of storylines, which have recently been used to look at the climatological processes underlying drought (Gessner et al., 2022; Shepherd et al., 2018; van der Wiel et al., 2021). Storylines can also be developed from hydrological and ecological data (Bastos et al., 2023) and be combined with qualitative social narratives to show the unfolding of the past or of plausible futures of the interconnected hydrological–ecological–social system. Additionally, earth system models, agent-based models, and system dynamics models are tools that explicitly allow for these interactions to be explored (see example in Bastos et al., 2023; de Ruiter and van Loon, 2022).
-
Observational data are critically needed, both for enhancing our process understanding of drought as a continuum in the hydrological–ecological–social system and for constraining interdisciplinary modelling tools. These data need to be multidisciplinary, covering different aspects of the system of systems, including its interactions and feedbacks, and collected already with interdisciplinarity studies in mind (Strang, 2009). They can be both qualitative and quantitative and can include proxies for processes that cannot easily be observed (Quandt, 2022; Rangecroft et al., 2021). These data should also be continuous and longitudinal, instead of event-based or project-limited. For example, longitudinal data can show long-term behavioural change in water consumption during and after drought (Sousa et al., 2022).
-
In this paper we focused on temporal scales; future research should also consider spatial scales, especially because some interactions take place at large spatial scales. Additionally, interactions across temporal and spatial scales are not often studied (Vanelli et al., 2022). We suggest that the appropriate temporal and spatial scales of analysis are determined for each piece of research specifically, dependent on the characteristics of the system under study. Relevant larger-scale processes can be considered boundary conditions for the system.
5.2 Practice outlook
Dealing with drought as a continuum in practice will require changes to how droughts and their impacts are monitored, modelled, forecasted, and managed and has implications for effective policy development. We suggest the following:
-
Drought monitoring needs to move from event-based to continuous monitoring, not only for hazard, but also for vulnerability and impacts. While several drought observatories still consider droughts to be single events or are only operational in specific seasons, there are some ongoing efforts to move to continuous monitoring that can serve as examples. For drought hazard, the European Drought Observatory (EDO, 2023), East Africa Drought Watch (EADW, 2023), and Rijkswaterstaat (see the Rhine River basin case study, Sect. S5) are moving towards continuous monitoring. For social and ecological drought impacts (Martínez-Vilalta and Lloret, 2016), the Drought Management Centre for Southeastern Europe (DMCSEE, 2023), Kenya National Drought Management Authority (NDMA, 2023), and Brazilian Drought Monitor (Walker et al., 2024) (see the northeast Brazil case study, Sect. S3) are examples where impacts are monitored on a continuous basis. Impacts on key ecological functions (plant productivity, water use, etc.) are monitored continuously and through multiple remote-sensing platforms and ecosystem monitoring networks with global or regional coverage (ICOS, AmeriFlux, FLUXNET, LTER). We are not aware of examples where vulnerability is monitored dynamically. We therefore recommend that key drought vulnerability indicators should also be monitored dynamically.
-
Monitoring of different systems needs to be combined to provide an overview of cascading effects between systems. The US Drought Monitor (USDM, 2023) is an example of combined drought hazard and impact monitoring that incorporates memory effects in different systems. The weekly drought map is a combination of physical drought indicators; drought impacts; field observations; and local insight from a network of more than 450 experts, including hydro-climatologists, ecologists, forest scientists, and relevant stakeholders. The maps are based on the information of the previous week and updated with new information. This approach builds in memory effects and explicitly includes drought recovery, both in the hazard and in the impacts. What is not explicitly included in these drought monitors, however, are vegetation responses, for example changes in transpiration, and management responses, such as increased irrigation, which can drive changes in the hydrological–ecological–social system in time. We suggest other drought monitors also include these memory effects in different systems and go one step further by also incorporating dynamic feedback.
-
Drought forecasting and early warning should be based on improved modelling tools that include memory and dynamic feedback (see “Scientific outlook”; Pulwarty and Sivakumar, 2014). Operational drought forecasting is currently limited to monthly precipitation and temperature forecasts, e.g. the USDM drought outlook (USDM, 2023) and Latin American drought forecast (SISSA, 2023), excluding memories in the hydrological system. Additionally, forecasting of drought impacts should be further developed to better anticipate societal and ecological impacts of drought. Some recent papers are taking steps in this direction, with longer-term processes, dynamic vulnerability, and memory effects being incorporated into impact forecasting (Boult et al., 2022; Busker et al., 2023). Operational drought impact forecasting is, however, still very limited (Shyrokaya et al., 2023). Only the East Africa Drought Watch (EADW, 2023) includes forage forecasts and is in the process of developing food and water security forecasts.
-
Drought management should be more prospective. Prospective management means that, instead of only proactively reducing risk for an upcoming event, exposure and vulnerability are reduced in the long term and maladaptation and the creation of new risks are avoided (UNDDR, 2021). Drought management will always need to include an element of short-term “early action” and crisis management to minimise unexpected impacts (Pulwarty and Sivakumar, 2014), but we support the notion that more attention should be paid to long-term adaptation and resilience building to avoid drought impacts and to plan the best strategies to reduce cascading effects within the hydrological–ecological–social system. Some recent approaches advocate for long-term drought resilience building and planning, e.g. the three-pillar approach suggested by the Integrated Drought Management Programme (IDMP, 2023), the related Drought Toolbox of UNCCD (2023), and the EPIC Response Framework of the World Bank (Browder et al., 2021). However, these efforts need to be accelerated and scaled up, and many established drought policies following this long-term approach have not been implemented.
-
Drought management should be more coordinated and integrated across actors and systems. The current approaches for governing drought, and water more generally, often contribute to a loss of social memory and maladaptation. This is because drought management is often highly siloed across different ministries or agencies due to its widespread effects on nearly all aspects of society (Bressers et al., 2016). This leads to significant fragmentation in the responsibility for managing drought across scales and sectors, which increases the complexity of governance (Teisman and Edelenbos, 2011). Consequently, calls for more collaborative and networked approaches to water management have become ubiquitous (Eberhard et al., 2017; Sabatier, 2005), though the implementation and effectiveness of such approaches are highly variable.
The examples mentioned in this section, drawn from real-world practices by organisations such as the IGAD Climate Prediction and Applications Centre (ICPAC) and IDMP, demonstrate successful strategies and highlight areas for improvement, serving as practical case studies for policymakers. In this way, they help reinforce the effectiveness of current policies, ensuring their continued relevance and implementation. Additionally, they provide a foundation for new policy initiatives by identifying best practices and innovative approaches that address emerging challenges in drought management.
These recommendations for science and practice will, we hope, contribute to the adoption of a changed perspective, where droughts are considered not in terms of a snapshot in time but rather in terms of a continuum of interrelated and dynamic hydrological–ecological–social processes. Considering drought to be a continuum will require a change in how droughts are monitored, modelled, and managed but will provide an opportunity for a more holistic and integrated approach to managing droughts and the impacts they have.
No original data were used to produce the figures and analysis in this paper.
The supplement related to this article is available online at: https://doi.org/10.5194/nhess-24-3173-2024-supplement.
AFVL, MicW, SaK, AM, JM, GGRN, RB, SiK, AS, and MLKW developed the original paper idea. AFVL, MicW, GV, EAK, SaK, and AM further developed its scope and structure. AFVL, SaK, AM, KEAH, FT, MinW, AS, MLKW, and MicW performed the literature review and wrote the sections, with contributions from RB, YD, PT, CAG, JM, CT, AB, FTdV, CHL, VN, GV, LC, EAK, ER, VA, and RT and support from IGP, TR, MG, MHB, JKLK, INS, RW, MLKW, and RS. CAG, ER, and AFVL led the case study collection. CAG, JPB, LC, MauG, MarG, RG, MI, SaK, EAK, MM, GGRN, INS, AFVL, RW, and MicW developed and analysed the case studies. AM and AFVL developed the figures, with input and feedback from FTdV, SaK, MLKW, MicW, MinW, LC, and GV. AFVL wrote the original draft with support from AM, EAK, FT, RT, GV, MLKW, MicW, MinW, and VN. All co-authors revised and edited the paper.
At least one of the (co-)authors is a member of the editorial board of Natural Hazards and Earth System Sciences. The peer-review process was guided by an independent editor, and the authors also have no other competing interests to declare.
Publisher's note: Copernicus Publications remains neutral with regard to jurisdictional claims made in the text, published maps, institutional affiliations, or any other geographical representation in this paper. While Copernicus Publications makes every effort to include appropriate place names, the final responsibility lies with the authors.
This article is part of the special issue “Drought, society, and ecosystems (NHESS/BG/GC/HESS inter-journal SI)”. It is a result of the Panta Rhei Drought in the Anthropocene workshop 2022, Uppsala, Sweden, 29–30 August 2022.
Elena Mondino was involved in earlier discussions related to this paper.
Anne F. Van Loon, Alessia Matanó, Ruben Weesie, Heidi D. Mendoza, and Marlies H. Barendrecht were funded by the European Union (ERC Starting Grant, PerfectSTORM, grant agreement no. 948601). Sarra Kchouk, Louise Cavalcante, and Germano G. Ribeiro Neto were supported by the Dutch Research Council (NWO) and the Interdisciplinary Research and Education Fund (INREF) of Wageningen University, the Netherlands (grant no. W07.30318.016). Minchao Wu was supported by the Swedish National Space Agency (SNSA; grant no. 2021-00111), the Swedish Research Council for Sustainable Development (FORMAS; grant no. 2022-00643), and Stiftelsen Oscar och Lili Lamms Minne (grant no. FO2022-0016). Viorica Nagavciuc was partially supported by a BMBF project and acknowledges funding via “Künstliche Intelligenz in der zivilen Sicherheitsforschung II” of the BMBF in the framework of “Forschung für die zivile Sicherheit” of the German Federal Government (https://www.sifo.de/sifo/de/home/home_node.html, last access: 7 September 2024). She was also partially supported by a grant of the Ministry of Research, Innovation and Digitalization, under “Romania's National Recovery and Resilience Plan” funded by the EU NextGenerationEU programme, project “Compound extreme events from a long-term perspective and their impact on forest growth dynamics” (CExForD) (grant no. 760074/23.05.2023; code 287/30.11.2022; within Pillar III, Component C9, Investment 8). Ana Bastos was funded by the European Union (ERC Starting Grant, ForExD, grant agreement no. 101039567). Margaret Garcia was supported by a National Science Foundation CAREER grant: Balancing Local and Systemic Resilience in the Western Water Network (CIS-1942370). Sina Khatami was jointly supported by a European Research Council (ERC) consolidator grant (grant no. 771678; HydroSocialExtremes), the EU-funded TRANSCEND project (grant no. 101084110), the Swedish Mannerfelt fond and Ahlmanns fond of the Bolin Centre for Climate Research (Sweden), and the HS&HK philanthropic fund (TO-53455-AUS, Australia). Micha Werner and Riccardo Biella were partially funded by the I-CISK project under the Horizon 2020 research and innovation programme (grant no. 101037293). Anastasiya Shyrokaya received funding from the European Union's Horizon 2020 research and innovation programme under Marie Skłodowska-Curie Actions (grant no. 956396; EDIPI Project). Khalid Hassaballah and Ileen Streefkerk were partially funded by the DOWN2EARTH project under the Horizon 2020 research and innovation programme (grant no. 869550).
Views and opinions expressed are however those of the authors only and do not necessarily reflect those of the funding agencies (e.g. the European Union or the European Research Council). Neither the European Union nor the granting authority can be held responsible for them.
This research has been supported by the European Union (ERC Starting Grant, PerfectSTORM (grant no. 948601); ERC Starting Grant, ForExD (grant no. 101039567); ERC Consolidator Grant, HydroSocialExtremes (grant no. 771678)), EU Horizon 2020 (grant nos. 101084110, 101037293, and 869550), EU Marie Skłodowska-Curie Actions (grant no. 956396), NWO and INREF (grant no. W07.30318.016), SNSA (grant no. 2021-00111), FORMAS (grant no. 2022-00643), Stiftelsen Oscar och Lili Lamms Minne (grant no. FO2022-0016), BMBF, NextGenerationEU (grant no. 760074/23.05.2023; code 287/30.11.2022), an NSF CAREER grant (CIS-1942370), Swedish Mannerfelt fond and Ahlmanns fond of the Bolin Centre for Climate Research (Sweden), and the HS&HK philanthropic fund (TO-53455-AUS, Australia).
This paper was edited by Floris van Ogtrop and reviewed by Ana Iglesias and Rene Orth.
Aanderud, Z. T., Jones, S. E., Fierer, N., and Lennon, J. T.: Resuscitation of the rare biosphere contributes to pulses of ecosystem activity, Front. Microbiol., 6, 24, https://doi.org/10.3389/fmicb.2015.00024, 2015.
Abatzoglou, J. T. and Williams, A. P.: Impact of anthropogenic climate change on wildfire across western US forests, P. Natl. Acad. Sci. USA, 113, 11770–11775, https://doi.org/10.1073/pnas.1607171113, 2016.
Acevedo, M., Pixley, K., Zinyengere, N., Meng, S., Tufan, H., Cichy, K., Bizikova, L., Isaacs, K., Ghezzi-Kopel, K., and Porciello, J.: A scoping review of adoption of climate-resilient crops by small-scale producers in low- and middle-income countries, Nat. Plants, 6, 1231–1241, https://doi.org/10.1038/s41477-020-00783-z, 2020.
Adams, H. D., Zeppel, M. J. B., Anderegg, W. R. L., Hartmann, H., Landhäusser, S. M., Tissue, D. T., Huxman, T. E., Hudson, P. J., Franz, T. E., Allen, C. D., Anderegg, L. D. L., Barron-Gafford, G. A., Beerling, D. J., Breshears, D. D., Brodribb, T. J., Bugmann, H., Cobb, R. C., Collins, A. D., Dickman, L. T., Duan, H., Ewers, B. E., Galiano, L., Galvez, D. A., Garcia-Forner, N., Gaylord, M. L., Germino, M. J., Gessler, A., Hacke, U. G., Hakamada, R., Hector, A., Jenkins, M. W., Kane, J. M., Kolb, T. E., Law, D. J., Lewis, J. D., Limousin, J.-M., Love, D. M., Macalady, A. K., Martínez-Vilalta, J., Mencuccini, M., Mitchell, P. J., Muss, J. D., O'Brien, M. J., O'Grady, A. P., Pangle, R. E., Pinkard, E. A., Piper, F. I., Plaut, J. A., Pockman, W. T., Quirk, J., Reinhardt, K., Ripullone, F., Ryan, M. G., Sala, A., Sevanto, S., Sperry, J. S., Vargas, R., Vennetier, M., Way, D. A., Xu, C., Yepez, E. A., and McDowell, N. G.: A multi-species synthesis of physiological mechanisms in drought-induced tree mortality, Nat. Ecol. Evol., 1, 1285–1291, https://doi.org/10.1038/s41559-017-0248-x, 2017.
Adla, S., Pande, S., Vico, G., Vora, S., Alam, M. F., Basel, B., Haeffner, M., and Sivapalan, M.: Place for sociohydrology in sustainable and climate-resilient agriculture: Review and ways forward, Cambridge Prism. Water, 1, e13, https://doi.org/10.1017/wat.2023.16, 2023.
Agee, J. K.: Fire Ecology of Pacific Northwest Forests, Island Press, Washington, D.C., ISBN 9781559632300, 1996.
Ahmed, M. M. M. and Abdalla, H. A.: Use of different nitrogen sources in the fattening of yearling sheep, Small Rumin. Res., 56, 39–45, https://doi.org/10.1016/j.smallrumres.2003.09.009, 2005.
Alessa, L. and Chapin, F. S.: Anthropogenic biomes: A key contribution to earth-system science, Trends Ecol. Evol., 23, 529–531, https://doi.org/10.1016/j.tree.2008.07.002, 2008.
Allen, C. D., Macalady, A. K., Chenchouni, H., Bachelet, D., McDowell, N., Vennetier, M., Kitzberger, T., Rigling, A., Breshears, D. D., Hogg, E. H. (Ted., Gonzalez, P., Fensham, R., Zhang, Z., Castro, J., Demidova, N., Lim, J. H., Allard, G., Running, S. W., Semerci, A., and Cobb, N.: A global overview of drought and heat-induced tree mortality reveals emerging climate change risks for forests, Forest. Ecol. Manage., 259, 660–684, https://doi.org/10.1016/j.foreco.2009.09.001, 2010.
Allen, C. D., Breshears, D. D., and McDowell, N.: On underestimation of global vulnerability to tree mortality and forest die-off|National Critical Zone Observatory, Ecosphere, 6, 129, https://doi.org/10.1890/ES15-00203.1, 2015.
Alvarez-Garreton, C., Pablo Boisier, J., Garreaud, R., Seibert, J., and Vis, M.: Progressive water deficits during multiyear droughts in basins with long hydrological memory in Chile, Hydrol. Earth Syst. Sci., 25, 429–446, https://doi.org/10.5194/hess-25-429-2021, 2021.
Alvarez-Garreton, C., Boisier, J. P., Billi, M., Lefort, I., Marinao, R., and Barría, P.: Protecting environmental flows to achieve long-term water security, J. Environ. Manage., 328, 116914, https://doi.org/10.1016/j.jenvman.2022.116914, 2023.
Alvarez-Garreton, C., Boisier, J. P., Garreaud, R., González, J., Rondanelli, R., Gayó, E., and Zambrano-Bigiarini, M.: HESS Opinions: The unsustainable use of groundwater conceals a “Day Zero”, Hydrol. Earth Syst. Sci., 28, 1605–1616, https://doi.org/10.5194/hess-28-1605-2024, 2024.
Amha, Y., Demissie, T., Amdihun, A., Otieno, V., Afiesimama, E., Murombedzi, J., and Radeny, M.: Unrelenting catastrophic droughts and successive failed rainy seasons in the Greater Horn of Africa What can we do better to protect millions of smallholder farmers and livestock keepers from extreme weather and climate crisis?, https://aiccra.cgiar.org/publications/unrelenting-catastrophic-droughts-and-successive-failed-rainy (last access: 5 July 2024), 2023.
Anderegg, W. R. L., Schwalm, C., Biondi, F., Camarero, J. J., Koch, G., Litvak, M., Ogle, K., Shaw, J. D., Shevliakova, E., Williams, A. P., Wolf, A., Ziaco, E., and Pacala, S.: Pervasive drought legacies in forest ecosystems and their implications for carbon cycle models, Science, 349, 528–532, https://doi.org/10.1126/science.aab1833, 2015.
Appels, W. M., Bogaart, P. W., and van der Zee, S. E. A. T. M.: Feedbacks Between Shallow Groundwater Dynamics and Surface Topography on Runoff Generation in Flat Fields, Water Resour. Res., 53, 10336–10353, https://doi.org/10.1002/2017WR020727, 2017.
Arheimer, B. and Lindström, G.: Climate impact on floods: Changes in high flows in Sweden in the past and the future (1911–2100), Hydrol. Earth Syst. Sci., 19, 771–784, https://doi.org/10.5194/hess-19-771-2015, 2015.
Armstrong, A., Dyer, E., Koehler, J., and Hope, R.: Intra-seasonal rainfall and piped water revenue variability in rural Africa, Global Environ. Change, 76, 102592, https://doi.org/10.1016/j.gloenvcha.2022.102592, 2022.
Ault, T. R., Mankin, J. S., Cook, B. I., and Smerdon, J. E.: Relative impacts of mitigation, temperature, and precipitation on 21st-century megadrought risk in the American Southwest, Sci. Adv., 2, 1–9, https://doi.org/10.1126/sciadv.1600873, 2016.
Bachmair, S., Kohn, I., and Stahl, K.: Exploring the link between drought indicators and impacts, Nat. Hazards Earth Syst. Sci., 15, 1381–1397, https://doi.org/10.5194/nhess-15-1381-2015, 2015.
Bachmair, S., Svensson, C., Hannaford, J., Barker, L. J., and Stahl, K.: A quantitative analysis to objectively appraise drought indicators and model drought impacts, Hydrol. Earth Syst. Sci., 20, 2589–2609, https://doi.org/10.5194/hess-20-2589-2016, 2016.
Bahmani, H. and Zhang, W.: Application of system thinking and factors interrelationship analysis to identify primary success factors of post-natural disaster recovery projects, Sustainability, 13, 3392, https://doi.org/10.3390/su13063392, 2021.
Bailey, R.: Managing Famine Risk: Linking Early Warning to Early Action, Chatham House, 1–114, https://www.chathamhouse.org/sites/default/files/public/Research/Energy,%20Environment%20and%20Development/0413r_earlywarnings_es.pdf (last access: 5 July 2024), 2013.
Barendrecht, M. H., Matanó, A., Mendoza, H. Weesie, R., Rohse, M. Koehler, J., De Ruiter, M. Garcia, M., Mazzoleni, M. Aerts, J. C. J. H., Ward, P., Di Baldassarre, G., and Van Loon, A.: Exploring drought-to-flood interactions and dynamics: A global case review, WIREs Water, 11, e1726, https://doi.org/10.1002/wat2.1726, 2024.
Barker, L. J., Hannaford, J., Chiverton, A., and Svensson, C.: From meteorological to hydrological drought using standardised indicators, Hydrol. Earth Syst. Sci., 20, 2483–2505, https://doi.org/10.5194/hess-20-2483-2016, 2016.
Bartholomeus, R. P., Mens, M., Muurling-van Geffen, S., Pot, W., Wanders, N., van Huijgevoort, M. H. J., van Loon, A. F., van Vliet, M. T. H., and van der Wiel, K.: Managing water across the flood–drought spectrum: Experiences from and challenges for the Netherlands, Cambridge Prism. Water, 1, e2, https://doi.org/10.1017/wat.2023.4, 2023.
Bastos, A., Ciais, P., Friedlingstein, P., Sitch, S., Pongratz, J., Fan, L., Wigneron, J. P., Weber, U., Reichstein, M., Fu, Z., Anthoni, P., Arneth, A., Haverd, V., Jain, A. K., Joetzjer, E., Knauer, J., Lienert, S., Loughran, T., McGuire, P. C., Tian, H., Viovy, N., and Zaehle, S.: Direct and seasonal legacy effects of the 2018 heat wave and drought on European ecosystem productivity, Sci. Adv., 6, eaba2724, https://doi.org/10.1126/sciadv.aba2724, 2020.
Bastos, A., Orth, R., Reichstein, M., Ciais, P., Viovy, N., Zaehle, S., Anthoni, P., Arneth, A., Gentine, P., Joetzjer, E., Lienert, S., Loughran, T., McGuire, P. C., Sungmin, O., Pongratz, J., and Sitch, S.: Vulnerability of European ecosystems to two compound dry and hot summers in 2018 and 2019, Earth Syst. Dynam., 12, 1015–1035, https://doi.org/10.5194/esd-12-1015-2021, 2021.
Bastos, A., Sippel, S., Frank, D., Mahecha, M. D., Zaehle, S., Zscheischler, J., and Reichstein, M.: A joint framework for studying compound ecoclimatic events, Nat. Rev. Earth Environ., 4, 333–350, https://doi.org/10.1038/s43017-023-00410-3, 2023.
Belesova, K., Agabiirwe, C. N., Zou, M., Phalkey, R., and Wilkinson, P.: Drought exposure as a risk factor for child undernutrition in low- and middle-income countries: A systematic review and assessment of empirical evidence, Environ. Int., 131, 104973, https://doi.org/10.1016/j.envint.2019.104973, 2019.
Biddle, J. C. and Baehler, K. J.: Breaking bad: When does polycentricity lead to maladaptation rather than adaptation?, Environ. Policy Gov., 29, 344–359, https://doi.org/10.1002/eet.1864, 2019.
Black, R. and Collyer, M.: Populations `trapped' at times of crisis, Forced Migr. Rev.-Cris., 45, 52–56, 2014.
Black, R., Adger, N., Arnell, N., Dercon, S., Geddes, A., and Thomas, D.: Migration and Global Environmental Change Future Challenges and Opportunities, London, https://assets.publishing.service.gov.uk/media/5a74b18840f0b61df4777b6c/11-1116-migration-and-global-environmental-change.pdf (last access: 5 July 2024), 2011.
Blauhut, V.: The triple complexity of drought risk analysis and its visualisation via mapping: a review across scales and sectors, Earth-Sci. Rev., 210, 103345, https://doi.org/10.1016/j.earscirev.2020.103345, 2020.
Blauhut, V., Stahl, K., Stagge, J. H., Tallaksen, L. M., De Stefano, L., and Vogt, J.: Estimating drought risk across Europe from reported drought impacts, drought indices, and vulnerability factors, Hydrol. Earth Syst. Sci., 20, 2779–2800, https://doi.org/10.5194/hess-20-2779-2016, 2016.
Blumenthal, D. M., Mueller, K. E., Kray, J. A., Ocheltree, T. W., Augustine, D. J., and Wilcox, K. R.: Traits link drought resistance with herbivore defence and plant economics in semi-arid grasslands: The central roles of phenology and leaf dry matter content, J. Ecol., 108, 2336–2351, https://doi.org/10.1111/1365-2745.13454, 2020.
Boisier, J. P., Rondanelli, R., Garreaud, R. D., Muñoz, F., Alvarez-Garreton, C., Cordero, R. R., Damiani, A., Gallardo, L., Garreaud, R. D., Lambert, F., Ramallo, C., Rojas, M., and Rondanelli, R.: Anthropogenic drying in central-southern Chile evidenced by long-term observations and climate model simulations, Elem. Sci. Anthr., 6, 74, https://doi.org/10.1525/elementa.328, 2018.
Botterill, L. and Hayes, M.: Drought triggers and declarations: Science and policy considerations for drought risk management, Nat. Hazards, 64, 139–151, https://doi.org/10.1007/s11069-012-0231-4, 2012.
Boult, V. L., Black, E., Saado Abdillahi, H., Bailey, M., Harris, C., Kilavi, M., Kniveton, D., MacLeod, D., Mwangi, E., Otieno, G., Rees, E., Rowhani, P., Taylor, O., and Todd, M. C.: Towards drought impact-based forecasting in a multi-hazard context, Clim. Risk Manage., 35, 100402, https://doi.org/10.1016/j.crm.2022.100402, 2022.
Bowles, T. M., Mooshammer, M., Socolar, Y., Calderón, F., Cavigelli, M. A., Culman, S. W., Deen, W., Drury, C. F., Garcia y Garcia, A., Gaudin, A. C. M., Harkcom, W. S., Lehman, R. M., Osborne, S. L., Robertson, G. P., Salerno, J., Schmer, M. R., Strock, J., and Grandy, A. S.: Long-Term Evidence Shows that Crop-Rotation Diversification Increases Agricultural Resilience to Adverse Growing Conditions in North America, One Earth, 2, 284–293, https://doi.org/10.1016/j.oneear.2020.02.007, 2020.
Brakkee, E., Van Huijgevoort, M. H. J., and Bartholomeus, R. P.: Improved understanding of regional groundwater drought development through time series modelling: The 2018–2019 drought in the Netherlands, Hydrol. Earth Syst. Sci., 26, 551–569, https://doi.org/10.5194/hess-26-551-2022, 2022.
Bressers, H., Bressers, N., and Larrue, C.: Governance for Drought Resilience Land and Water Drought Management in Europe, Springer, https://doi.org/10.1007/978-3-319-29671-5, 2016.
Browder, G., Nunez Sanchez, Ana Jongman, B., Engle, N., van Beek, E., Errea, C., and Melissa Hodgson, S.: An EPIC Response: Innovative Governance for Flood and Drought Risk Management, Washington, D.C., https://reliefweb.int/report/world/epic-response-innovative-governance-flood-and-drought-risk-management?gad_source=1&gclid=CjwKCAjwreW2BhBhEi wAavLwfI9BeMnr9HJ7ess_-T0edcpii9N4LykMGTBMPyFjlh rTMaHAx5uHVhoCj00QAvD_BwE (last access: 5 July 2024), 2021.
Brunner, M. I., Slater, L., Tallaksen, L. M., and Clark, M.: Challenges in modeling and predicting floods and droughts: A review, Wiley Interdisciplin. Rev. Water, 8, 1–32, https://doi.org/10.1002/wat2.1520, 2021.
Busker, T., de Moel, H., van den Hurk, B., and Aerts, J. C. J. H.: Impact-based seasonal rainfall forecasting to trigger early action for droughts, Sci. Total Environ., 898, 165506, https://doi.org/10.1016/j.scitotenv.2023.165506, 2023.
Buttler, A., Mariotte, P., Meisser, M., Guillaume, T., Signarbieux, C., Vitra, A., Preux, S., Mercier, G., Quezada, J., Bragazza, L., and Gavazov, K.: Drought-induced decline of productivity in the dominant grassland species Lolium perenne L. depends on soil type and prevailing climatic conditions, Soil Biol. Biochem., 132, 47–57, https://doi.org/10.1016/j.soilbio.2019.01.026, 2019.
Carmichael, T. and Hadžikadić, M.: The Fundamentals of Complex Adaptive Systems BT – Complex Adaptive Systems: Views from the Physical, Natural, and Social Sciences, edited by: Carmichael, T., Collins, A. J., and Hadžikadić, M., Springer International Publishing, Cham, 1–16, https://doi.org/10.1007/978-3-030-20309-2_1, 2019.
Carse, A.: An infrastructural event: Making sense of Panama's drought, Water Altern., 10, 888–909, 2017.
Charnley, G. E. C., Kelman, I., Green, N., Hinsley, W., Gaythorpe, K. A. M., and Murray, K. A.: Exploring relationships between drought and epidemic cholera in Africa using generalised linear models, BMC Infect. Dis., 21, 1–12, https://doi.org/10.1186/s12879-021-06856-4, 2021.
Choat, B., Brodribb, T. J., Brodersen, C. R., Duursma, R. A., López, R., and Medlyn, B. E.: Triggers of tree mortality under drought, Nature, 558, 531–539, https://doi.org/10.1038/s41586-018-0240-x, 2018.
Christian, J. I., Basara, J. B., Hunt, E. D., Otkin, J. A., Furtado, J. C., Mishra, V., Xiao, X., and Randall, R. M.: Global distribution, trends, and drivers of flash drought occurrence, Nat. Commun., 12, 1–11, https://doi.org/10.1038/s41467-021-26692-z, 2021.
Colombo, N., Guyennon, N., Valt, M., Salerno, F., Godone, D., Cianfarra, P., Freppaz, M., Maugeri, M., Manara, V., Acquaotta, F., Petrangeli, A. B., and Romano, E.: Unprecedented snow-drought conditions in the Italian Alps during the early 2020s, Environ. Res. Lett., 18, 074014, https://doi.org/10.1088/1748-9326/acdb88, 2023.
Cook, B. I., Seager, R., and Smerdon, J. E.: The worst North American drought year of the last millennium: 1934, Geophys. Res. Lett., 41, 7298–7305, https://doi.org/10.1002/2014GL061661, 2014.
Cook, B. I., Smerdon, J. E., Cook, E. R., Williams, A. P., Anchukaitis, K. J., Mankin, J. S., Allen, K., Andreu-Hayles, L., Ault, T. R., Belmecheri, S., Coats, S., Coulthard, B., Fosu, B., Grierson, P., Griffin, D., Herrera, D. A., Ionita, M., Lehner, F., Leland, C., Marvel, K., Morales, M. S., Mishra, V., Ngoma, J., Nguyen, H. T. T., O'Donnell, A., Palmer, J., Rao, M. P., Rodriguez-Caton, M., Seager, R., Stahle, D. W., Stevenson, S., Thapa, U. K., Varuolo-Clarke, A. M., and Wise, E. K.: Megadroughts in the Common Era and the Anthropocene, Nat. Rev. Earth Environ., 3, 741–757, https://doi.org/10.1038/s43017-022-00329-1, 2022.
Cranko Page, J., De Kauwe, M. G., Abramowitz, G., and Pitman, A. J.: Non-Stationary Lags and Legacies in Ecosystem Flux Response to Antecedent Rainfall, J. Geophys. Res.-Biogeosci., 128, e2022JG007144, https://doi.org/10.1029/2022JG007144, 2023.
Crausbay, S. D., Betancourt, J., Bradford, J., Cartwright, J., Dennison, W. C., Dunham, J., Enquist, C. A. F., Frazier, A. G., Hall, K. R., Littell, J. S., Luce, C. H., Palmer, R., Ramirez, A. R., Rangwala, I., Thompson, L., Walsh, B. M., and Carter, S.: Unfamiliar Territory: Emerging Themes for Ecological Drought Research and Management, One Earth, 3, 337–353, https://doi.org/10.1016/j.oneear.2020.08.019, 2020.
Dean, J. and Stain, H. J.: The Impact of Drought on the Emotional Well-Being of Children and Adolescents in Rural and Remote New South Wales, J. Rural Heal., 23, 356–364, https://doi.org/10.1111/j.1748-0361.2007.00113.x, 2007.
de Brito, M. M.: Compound and cascading drought impacts do not happen by chance: A proposal to quantify their relationships, Sci. Total Environ., 778, 146236, https://doi.org/10.1016/j.scitotenv.2021.146236, 2021.
de Brito, M. M., Kuhlicke, C., and Marx, A.: Near-real-time drought impact assessment: A text mining approach on the 2018/19 drought in Germany, Environ. Res. Lett., 15, 1040a9, https://doi.org/10.1088/1748-9326/aba4ca, 2020.
De Lavenne, A., Andréassian, V., Crochemore, L., Lindström, G., and Arheimer, B.: Quantifying multi-year hydrological memory with Catchment Forgetting Curves, Hydrol. Earth Syst. Sci., 26, 2715–2732, https://doi.org/10.5194/hess-26-2715-2022, 2022.
Delgado-Serrano, M., Oteros-Rozas, E., Vanwildemeersch, P., Guerrero, C. O., London, S., and Escalante, R.: Local perceptions on social-ecological dynamics in Latin America in three community-based natural resource management systems, Ecol. Soc., 20, 24, https://doi.org/10.5751/ES-07965-200424, 2015.
De Long, J. R., Semchenko, M., Pritchard, W. J., Cordero, I., Fry, E. L., Jackson, B. G., Kurnosova, K., Ostle, N. J., Johnson, D., Baggs, E. M., and Bardgett, R. D.: Drought soil legacy overrides maternal effects on plant growth, Funct. Ecol., 33, 1400–1410, https://doi.org/10.1111/1365-2435.13341, 2019.
De Polt, K., Ward, P. J., de Ruiter, M., Bogdanovich, E., Reichstein, M., Frank, D., and Orth, R.: Quantifying impact-relevant heatwave durations, Environ. Res. Lett., 18, 104005, https://doi.org/10.1088/1748-9326/acf05e, 2023.
de Ruiter, M. C. and van Loon, A. F.: The challenges of dynamic vulnerability and how to assess it, iScience, 25, 104720, https://doi.org/10.1016/j.isci.2022.104720, 2022.
Deslatte, A., Koebele, E. A., Bartels, L., Wiechman, A., Vicario, S. A., Coughlin, C., and Rybolt, D.: Institutions, Voids, and Dependencies: Tracing the Designs and Robustness of Urban Water Systems, Int. Rev. Publ. Policy, 5, 1–25, https://doi.org/10.4000/irpp.3455, 2023.
de Vries, F., Lau, J., Hawkes, C., and Semchenko, M.: Plant–soil feedback under drought: does history shape the future?, Trends Ecol. Evol., 38, 708–718, https://doi.org/10.1016/j.tree.2023.03.001, 2023.
de Vries, F. T., Brown, C., and Stevens, C. J.: Grassland species root response to drought: consequences for soil carbon and nitrogen availability, Plant Soil, 409, 297–312, https://doi.org/10.1007/s11104-016-2964-4, 2016.
de Vries, F. T., Griffiths, R. I., Bailey, M., Craig, H., Girlanda, M., Gweon, H. S., Hallin, S., Kaisermann, A., Keith, A. M., Kretzschmar, M., Lemanceau, P., Lumini, E., Mason, K. E., Oliver, A., Ostle, N., Prosser, J. I., Thion, C., Thomson, B., and Bardgett, R. D.: Soil bacterial networks are less stable under drought than fungal networks, Nat. Commun., 9, 3033, https://doi.org/10.1038/s41467-018-05516-7, 2018.
de Vries, F. T., Williams, A., Stringer, F., Willcocks, R., McEwing, R., Langridge, H., and Straathof, A. L.: Changes in root-exudate-induced respiration reveal a novel mechanism through which drought affects ecosystem carbon cycling, New Phytol., 224, 132–145, https://doi.org/10.1111/nph.16001, 2019.
Di Baldassarre, G., Viglione, A., Carr, G., Kuil, L., Salinas, J. L., and Blöschl, G.: Socio-hydrology: Conceptualising human-flood interactions, Hydrol. Earth Syst. Sci., 17, 3295–3303, https://doi.org/10.5194/hess-17-3295-2013, 2013.
Di Baldassarre, G., Wanders, N., AghaKouchak, A., Kuil, L., Rangecroft, S., Veldkamp, T. I. E., Garcia, M., van Oel, P. R., Breinl, K., and Van Loon, A. F.: Water shortages worsened by reservoir effects, Nat. Sustainabil., 1, 617–622, https://doi.org/10.1038/s41893-018-0159-0, 2018.
Ding, Y., Hayes, M. J., and Widhalm, M.: Measuring economic impacts of drought: a review and discussion, Disast. Prev. Manage., 20, 434–446, 2011.
DMCSEE: Drought Management Centre for Southeastern Europe, Drought Manag. Cent. South East Eur., https://dmcsee.org/ (last access: 21 January 2024), 2023.
Du, Y., Clemenzi, I., and Pechlivanidis, I. G.: Hydrological regimes explain the seasonal predictability of streamflow extremes, Environ. Res. Lett., 18, 094060, https://doi.org/10.1088/1748-9326/acf678, 2023.
EADW: East Africa Drought Watch Monitor drought conditions in East Africa, IGAD Clim. Predict. Appl. Cent., https://droughtwatch.icpac.net/ (last (access: 12 July 2023), 2023.
Eberhard, A., Gratwick, K., Morella, E., and Antmann, P.: Correction: Accelerating investments in power in sub-Saharan Africa, Nat. Energy, 2, 17044, https://doi.org/10.1038/nenergy.2017.44, 2017.
EDO: European Drought Observatory, Eur. Drought Obs., https://edo.jrc.ec.europa.eu/ (last access: 21 January 2024), 2023.
Eltahir, E. A. B. and Yeh, P. J. F.: On the asymmetric response of aquifer water level to floods and droughts in Illinois, Water Resour. Res., 35, 1199–1217, https://doi.org/10.1029/1998WR900071, 1999.
EM-DAT: Inventorying hazards & disasters worldwide since 1988, EM-DAT https://www.emdat.be/ (last access: 7 December 2023), 2023.
Enqvist, J., Ziervogel, G., Metelerkamp, L., van Breda, J., Dondi, N., Lusithi, T., Mdunyelwa, A., Mgwigwi, Z., Mhlalisi, M., Myeza, S., Nomela, G., October, A., Rangana, W., and Yalabi, M.: Informality and water justice: community perspectives on water issues in Cape Town's low-income neighbourhoods, Int. J. Water Resour. Dev., 38, 108–129, https://doi.org/10.1080/07900627.2020.1841605, 2022.
Enqvist, J. P. and Ziervogel, G.: Water governance and justice in Cape Town: An overview, Wiley Interdisciplin. Rev.: Water, 6, e1354, https://doi.org/10.1002/wat2.1354, 2019.
Estrela, T. and Sancho, T. A.: Drought management policies in Spain and the european union: From traditional emergency actions to drought management plans, Water Policy, 18, 153–176, https://doi.org/10.2166/wp.2016.018, 2016.
EUCRA: European Climate Risk Assessment, Eur. Clim. Risk Assess., https://www.eea.europa.eu/en/about/who-we-are/projects-and-cooperation-agreements/european-climate-risk-assessment (last access: 21 January 2024), 2024.
Falco, C., Galeotti, M., and Olper, A.: Climate change and migration: Is agriculture the main channel?, Global Environ. Change, 59, 101995, https://doi.org/10.1016/j.gloenvcha.2019.101995, 2019.
Fatulová, E.: Guidelines for preparation of the Drought Management Plans, https://www.gwp.org/globalassets/global/gwp-cee_images/idmp-draft-guidelines-15-september-2014.pdf (last access: 5 July 2024), 2014.
Fettig, C. J., Mortenson, L. A., Bulaon, B. M., and Foulk, P. B.: Tree mortality following drought in the central and southern Sierra Nevada, California, U.S., Forest Ecol. Manage., 432, 164–178, https://doi.org/10.1016/j.foreco.2018.09.006, 2019.
Flach, M., Sippel, S., Gans, F., Bastos, A., Brenning, A., Reichstein, M., and Mahecha, M. D.: Contrasting biosphere responses to hydrometeorological extremes: revisiting the 2010 western Russian heatwave, Biogeosciences, 15, 6067–6085, https://doi.org/10.5194/bg-15-6067-2018, 2018.
Fowler, K., Knoben, W., Peel, M., Peterson, T., Ryu, D., Saft, M., Seo, K. W., and Western, A.: Many Commonly Used Rainfall-Runoff Models Lack Long, Slow Dynamics: Implications for Runoff Projections, Water Resour. Res., 56, e2019WR02528, https://doi.org/10.1029/2019WR025286, 2020.
Fu, X., Svoboda, M., Tang, Z., Dai, Z., and Wu, J.: An overview of US state drought plans: crisis or risk management?, Nat. Hazards, 69, 1607–1627, https://doi.org/10.1007/s11069-013-0766-z, 2013.
Fuchs, E. H., King, J. P., and Carroll, K. C.: Quantifying Disconnection of Groundwater From Managed-Ephemeral Surface Water During Drought and Conjunctive Agricultural Use, Water Resour. Res., 55, 5871–5890, https://doi.org/10.1029/2019WR024941, 2019.
Fuchslueger, L., Bahn, M., Hasibeder, R., Kienzl, S., Fritz, K., Schmitt, M., Watzka, M., and Richter, A.: Drought history affects grassland plant and microbial carbon turnover during and after a subsequent drought event, J. Ecol., 104, 1453–1465, https://doi.org/10.1111/1365-2745.12593, 2016.
Galleguillos, M., Gimeno, F., Puelma, C., Zambrano-Bigiarini, M., Lara, A., and Rojas, M.: Disentangling the effect of future land use strategies and climate change on streamflow in a Mediterranean catchment dominated by tree plantations, J. Hydrol., 595, 126047, https://doi.org/10.1016/j.jhydrol.2021.126047, 2021.
Garcia, M., Ridolfi, E., and Di Baldassarre, G.: The interplay between reservoir storage and operating rules under evolving conditions, J. Hydrol., 590, 125270, https://doi.org/10.1016/j.jhydrol.2020.125270, 2020.
Garcia, M., Yu, D., Park, S., Yousefi Bahambari, P., Mohajer Iravanloo, B., and Sivapalan, M.: Weathering water extremes and cognitive biases in a changing climate, Water Secur., 15, 100110, https://doi.org/10.1016/j.wasec.2022.100110, 2022.
Gebrehiwot, T. and van der Veen, A.: Farmers' drought experience, risk perceptions, and behavioural intentions for adaptation: evidence from Ethiopia, Clim. Dev., 13, 493–502, https://doi.org/10.1080/17565529.2020.1806776, 2021.
Gessner, C., Fischer, E. M., Beyerle, U., and Knutti, R.: Multi-year drought storylines for Europe and North America from an iteratively perturbed global climate model, Weather Clim. Extrem., 38, 100512, https://doi.org/10.1016/j.wace.2022.100512, 2022.
Gevaert, A. I., Veldkamp, T. I. E., and Ward, P. J.: The effect of climate type on timescales of drought propagation in an ensemble of global hydrological models, Hydrol. Earth Syst. Sci., 22, 4649–4665, https://doi.org/10.5194/hess-22-4649-2018, 2018.
Ghajarnia, N., Kalantari, Z., Orth, R., and Destouni, G.: Close co-variation between soil moisture and runoff emerging from multi-catchment data across Europe, Sci. Rep., 10, 4817, https://doi.org/10.1038/s41598-020-61621-y, 2020.
Gliesch, M., Hinojosa Sanchez, L., Jongepier, E., Martin, C., Hu, Y., Tietema, A., and de Vries, F. T.: Heathland management affects soil response to drought, J. Appl. Ecol., 61, 1372–1384, https://doi.org/10.1111/1365-2664.14641, 2024.
Gouveia, C. M., Trigo, R. M., Beguería, S., and Vicente-Serrano, S. M.: Drought impacts on vegetation activity in the Mediterranean region: An assessment using remote sensing data and multi-scale drought indicators, Global Planet. Change, 151, 15–27, https://doi.org/10.1016/j.gloplacha.2016.06.011, 2017.
Griffin-Nolan, R. J., Carroll, C. J. W., Denton, E. M., Johnston, M. K., Collins, S. L., Smith, M. D., and Knapp, A. K.: Legacy effects of a regional drought on aboveground net primary production in six central US grasslands, Plant Ecol., 219, 505–515, https://doi.org/10.1007/s11258-018-0813-7, 2018.
Griffin-Nolan, R. J., Blumenthal, D. M., Collins, S. L., Farkas, T. E., Hoffman, A. M., Mueller, K. E., Ocheltree, T. W., Smith, M. D., Whitney, K. D., and Knapp, A. K.: Shifts in plant functional composition following long-term drought in grasslands, J. Ecol., 107, 2133–2148, https://doi.org/10.1111/1365-2745.13252, 2019.
Griffiths, H. M. and Tooth, S.: Remembering and forgetting floods and droughts: lessons from the Welsh colony in Patagonia, Cult. Geogr., 28, 341–361, https://doi.org/10.1177/1474474020963135, 2021.
Grossiord, C., Buckley, T. N., Cernusak, L. A., Novick, K. A., Poulter, B., Siegwolf, R. T. W., Sperry, J. S., and McDowell, N. G.: Plant responses to rising vapor pressure deficit, New Phytol., 226, 1150–1566, https://doi.org/10.1111/nph.16485, 2020.
Gu, H., Xu, Y. P., Liu, L., Xie, J., Wang, L., Pan, S., and Guo, Y.: Seasonal catchment memory of high mountain rivers in the Tibetan Plateau, Nat. Commun., 14, 3173, https://doi.org/10.1038/s41467-023-38966-9, 2023.
Gunderson, L. and Holling, C.: Panarchy: Understanding Transformations In Human And Natural Systems, Island Press, https://doi.org/10.1016/S0006-3207(03)00041-7, 2002.
GWP: Integrated water resources management in Eastern Africa, Glob. Water Partnersh., https://www.gwp.org/globalassets/global/toolbox/publications/technical-focus-papers/p1238_gwp_tfp_ea_121015_web.pdf (last access: 5 July 2024), 2015.
GWP CEE: Integrated Drought Management Programme in Central and Eastern Europe, GWP CEE – Glob. Water Partnersh. Cent. East. Eur., Available https://www.droughtmanagement.info/literature/idmp-cee_compendium_en.pdf (last access: 5 July 2024), 2014.
Haasnoot, M., Kwakkel, J. H., Walker, W. E., and ter Maat, J.: Dynamic adaptive policy pathways: A method for crafting robust decisions for a deeply uncertain world, Global Environ. Change, 23, 485–498, https://doi.org/10.1016/j.gloenvcha.2012.12.006, 2013.
Haberstroh, S. and Werner, C.: The role of species interactions for forest resilience to drought, Plant Biol., 24, 1098–1107, https://doi.org/10.1111/plb.13415, 2022.
Hagenlocher, M., Meza, I., Anderson, C. C., Min, A., Renaud, F. G., Walz, Y., Siebert, S., and Sebesvari, Z.: Drought vulnerability and risk assessments: State of the art, persistent gaps, and research agenda, Environ. Res. Lett., 14, 083002, https://doi.org/10.1088/1748-9326/ab225d, 2019.
Hagenlocher, M., Naumann, G., Meza, I., Blauhut, V., Cotti, D., Döll, P., Ehlert, K., Gaupp, F., Van Loon, A. F., Marengo, J. A., Rossi, L., Sabino Siemons, A. S., Siebert, S., Tsehayu, A. T., Toreti, A., Tsegai, D., Vera, C., Vogt, J., and Wens, M.: Tackling Growing Drought Risks – The Need https://doi.org/10.1029/2023EF003857, 2023.
Hahn, C., Lüscher, A., Ernst-Hasler, S., Suter, M., and Kahmen, A.: Timing of drought in the growing season and strong legacy effects determine the annual productivity of temperate grasses in a changing climate, Biogeosciences, 18, 585–604, https://doi.org/10.5194/bg-18-585-2021, 2021.
Hall, J. W. and Leng, G.: Can we calculate drought risk … and do we need to?, Wiley Interdisciplin. Rev.: Water, 6, 2–5, https://doi.org/10.1002/wat2.1349, 2019.
Hammond, W. M., Yu, K., Wilson, L. A., Will, R. E., Anderegg, W. R. L., and Adams, H. D.: Dead or dying? Quantifying the point of no return from hydraulic failure in drought-induced tree mortality, New Phytol., 223, 1834–1843, https://doi.org/10.1111/nph.15922, 2019.
Hantson, S., Arneth, A., Harrison, S. P., Kelley, D. I., Prentice, I. C., Rabin, S. S., Archibald, S., Mouillot, F., Arnold, S. R., Artaxo, P., Bachelet, D., Ciais, P., Forrest, M., Friedlingstein, P., Hickler, T., Kaplan, J. O., Kloster, S., Knorr, W., Lasslop, G., Li, F., Mangeon, S., Melton, J. R., Meyn, A., Sitch, S., Spessa, A., van der Werf, G. R., Voulgarakis, A., and Yue, C.: The status and challenge of global fire modelling, Biogeosciences, 13, 3359–3375, https://doi.org/10.5194/bg-13-3359-2016, 2016.
Hartmann, H., Bahn, M., Carbone, M., and Richardson, A. D.: Plant carbon allocation in a changing world – challenges and progress: introduction to a Virtual Issue on carbon allocation: Introduction to a virtual issue on carbon allocation, New Phytol., 227, 981–988, https://doi.org/10.1111/nph.16757, 2020.
Hessburg, P. F. and Agee, J. K.: An environmental narrative of Inland Northwest United States forests, 1800–2000, Forest Ecol. Manage., 178, 23–59, https://doi.org/10.1016/S0378-1127(03)00052-5, 2003.
Hisdal, H., Tallaksen, L. M., Peters, E., Stahl, K., and Zaidman, M.: Drought event definition, Arid. Tech. Rep., https://www.hydrology.uni-freiburg.de/forsch/aride/navigation/publications/pdfs/aride-techrep6.pdf (last access: 5 July 2024), 2000.
Hoek van Dijke, A. J., Herold, M., Mallick, K., Benedict, I., Machwitz, M., Schlerf, M., Pranindita, A., Theeuwen, J. J. E., Bastin, J.-F., and Teuling, A. J.: Shifts in regional water availability due to global tree restoration, Nat. Geosci., 15, 363–368, https://doi.org/10.1038/s41561-022-00935-0, 2022.
Holden, Z. A., Swanson, A., Luce, C. H., Jolly, W. M., Maneta, M., Oyler, J. W., Warren, D. A., Parsons, R., and Affleck, D.: Decreasing fire season precipitation increased recent western US forest wildfire activity, P. Natl. Acad. Sci. USA, 115, E8349–E8357, https://doi.org/10.1073/pnas.1802316115, 2018.
Howden, M., Schroeter, S., Crimp, S., and Hanigan, I.: The changing roles of science in managing Australian droughts: An agricultural perspective, Weather Clim. Extrem., 3, 80–89, https://doi.org/10.1016/j.wace.2014.04.006, 2014.
Hsiao, T. C.: Plant Responses to Water Stress, Annu. Rev. Plant Physiol., 24, 519–570, https://doi.org/10.1146/annurev.pp.24.060173.002511, 1973.
Huang, L., Zhou, P., Cheng, L., and Liu, Z.: Dynamic drought recovery patterns over the Yangtze River Basin, Catena, 201, 105194, https://doi.org/10.1016/j.catena.2021.105194, 2021.
Hurlbert, M. and Gupta, J.: Adaptive Governance, Uncertainty, and Risk: Policy Framing and Responses to Climate Change, Drought, and Flood, Risk Anal., 36, 339–356, https://doi.org/10.1111/risa.12510, 2016.
Hyland, M. and Russ, J.: Water as destiny – The long-term impacts of drought in sub-Saharan Africa, World Dev., 115, 30–45, https://doi.org/10.1016/j.worlddev.2018.11.002, 2019.
IDMP: Integrated Drought Management Programme, Integr. Drought Manag. Program, https://www.droughtmanagement.info/ (last access: 21 January 2024), 2023.
Iglesias, A., Garrote, L., and Cancelliere, A.: Guidelines to Develop Drought Management Plans, in: Coping with Drought Risk in Agriculture and Water Supply Systems, edited by: Iglesias, A., Cancelliere, A., Wilhite, D. A., Garrote, L., and Cubillo, F., Advances in Natural and Technological Hazards Research, vol. 26, Springer, Dordrecht, https://doi.org/10.1007/978-1-4020-9045-5_5, 2009.
Iizumi, T., Shin, Y., Kim, W., Kim, M., and Choi, J.: Global crop yield forecasting using seasonal climate information from a multi-model ensemble, Clim. Serv., 11, 13–23, https://doi.org/10.1016/j.cliser.2018.06.003, 2018.
Ionita, M. and Nagavciuc, V.: Forecasting low flow conditions months in advance through teleconnection patterns, with a special focus on summer 2018, Sci. Rep., 10, 13258, https://doi.org/10.1038/s41598-020-70060-8, 2020.
Ionita, M. and Nagavciuc, V.: Changes in drought features at the European level over the last 120 years, Nat. Hazards Earth Syst. Sci., 21, 1685–1701, https://doi.org/10.5194/nhess-21-1685-2021, 2021.
Ionita, M., Dima, M., Nagavciuc, V., Scholz, P., and Lohmann, G.: Past megadroughts in central Europe were longer, more severe and less warm than modern droughts, Commun. Earth Environ., 2, 61, https://doi.org/10.1038/s43247-021-00130-w, 2021.
IPCC: Climate Change 2014: Impacts, Adaptation, and Vulnerability. Part A: Global and Sectoral Aspects, in: Contribution of Working Group II to the Fifth Assessment Report of the Intergovernmental Panel on Climate Change, edited by: Field, C. B., Barros, V. R., Dokken, D. J., Mach, K. J., Mastrandrea, M. D., Bilir, T. E., Chatterjee, M., Ebi, K. L., Estrada, Y. O., Genova, R. C., Girma, B., Kissel, E. S., Levy, A. N., MacCracken, S., Mastrandrea, P. R., and White, L. L., Cambridge University Press, Cambridge, UK and New York, NY, USA, https://www.ipcc.ch/site/assets/uploads/2018/02/WGIIAR5-FrontMatterA_FINAL.pdf (last access: 5 July 2024), 2014.
Ison, R. L., Maiteny, P. T., and Carr, S.: Systems methodologies for sustainable natural resources research and development, Agric. Syst., 55, 257–272, https://doi.org/10.1016/S0308-521X(97)00010-3, 1997.
Jackson, M.: Critical Systems Thinking and the Management of Complexity, John Wiley & Sons Ltd., ISBN 978-1119118374, 2019.
Jackson, M. C., Pawar, S., and Woodward, G.: The Temporal Dynamics of Multiple Stressor Effects: From Individuals to Ecosystems, Trends Ecol. Evol., 36, 402–410, https://doi.org/10.1016/j.tree.2021.01.005, 2021.
Jacobsen, A. L., Pratt, R. B., Davis, S. D., and Ewers, F. W.: Comparative community physiology: nonconvergence in water relations among three semi-arid shrub communities, New Phytol., 180, 100–113, https://doi.org/10.1111/j.1469-8137.2008.02554.x, 2008.
Juhola, S., Glaas, E., Linnér, B.-O., and Neset, T.-S.: Redefining maladaptation, Environ. Sci. Policy, 55, 135–140, https://doi.org/10.1016/j.envsci.2015.09.014, 2016.
Kannenberg, S. A., Novick, K. A., Alexander, M. R., Maxwell, J. T., Moore, D. J. P., Phillips, R. P., and Anderegg, W. R. L.: Linking drought legacy effects across scales: From leaves to tree rings to ecosystems, Global Change Biol., 25, 2978–2992, https://doi.org/10.1111/gcb.14710, 2019.
Kannenberg, S. A., Schwalm, C. R., and Anderegg, W. R. L.: Ghosts of the past: how drought legacy effects shape forest functioning and carbon cycling, Ecol. Lett., 23, 891–901, https://doi.org/10.1111/ele.13485, 2020.
Karambelkar, S. and Gerlak, A. K.: Collaborative governance and stakeholder participation in the colorado river basin: An examination of patterns of inclusion and exclusion, Nat. Resour. J., 60, 1–47, 2020.
Kchouk, S., Melsen, L. A., Walker, D. W., and van Oel, P. R.: A geography of drought indices: mismatch between indicators of drought and its impacts on water and food securities, Nat. Hazards Earth Syst. Sci., 22, 323–344, https://doi.org/10.5194/nhess-22-323-2022, 2022.
Kchouk, S., Neto, G. R., Melsen, L. A., Walker, D. W., Cavalcante, L., Gondim, R., and van Oel, P. R.: Drought-impacted communities in social-ecological systems: Exploration of different system states in Northeast Brazil, Int. J. Disast. Risk Reduct., 97, 104026, https://doi.org/10.1016/j.ijdrr.2023.104026, 2023.
Keshavarz, M., Karami, E., and Vanclay, F.: The social experience of drought in rural Iran, Land Use Policy, 30, 120–129, https://doi.org/10.1016/j.landusepol.2012.03.003, 2013.
Khanal, S., Lutz, A. F., Immerzeel, W. W., de Vries, H., Wanders, N., and van den Hurk, B.: The impact of meteorological and hydrological memory on compound peak flows in the Rhine river basin, Atmosphere, 10, 171, https://doi.org/10.3390/atmos10040171, 2019.
Kiem, A. S., Johnson, F., Westra, S., van Dijk, A., Evans, J. P., O'Donnell, A., Rouillard, A., Barr, C., Tyler, J., Thyer, M., Jakob, D., Woldemeskel, F., Sivakumar, B., and Mehrotra, R.: Natural hazards in Australia: droughts, Climatic Change, 139, 37–54, https://doi.org/10.1007/s10584-016-1798-7, 2016.
King-Okumu, C., Tsegai, D., Pandey, R. P., and Rees, G.: Less to lose? Drought impact and vulnerability assessment in disadvantaged regions, Water, 12, 1136, https://doi.org/10.3390/w12041136, 2020.
Kleine, L., Tetzlaff, D., Smith, A., Goldhammer, T., and Soulsby, C.: Using isotopes to understand landscape-scale connectivity in a groundwater-dominated, lowland catchment under drought conditions, Hydrol. Process., 35, e14197, https://doi.org/10.1002/hyp.14197, 2021.
KMNI: Droogtemonitor, R. Netherlands Meteorol. Inst., https://www.knmi.nl/nederland-nu/klimatologie/droogtemonitor (last access: 20 January 2024), 2023.
Koebele, E. A., Crow, D. A., and Albright, E. A.: Building Resilience during Recovery: Lessons from Colorado's Watershed Resilience Pilot Program, Environ. Manage., 66, 1–15, https://doi.org/10.1007/s00267-020-01296-3, 2020.
Kolb, T. E., Fettig, C. J., Ayres, M. P., Bentz, B. J., Hicke, J. A., Mathiasen, R., Stewart, J. E., and Weed, A. S.: Observed and anticipated impacts of drought on forest insects and diseases in the United States, Forest Ecol. Manage., 380, 321–334, https://doi.org/10.1016/j.foreco.2016.04.051, 2016.
Konar, M., Garcia, M., Sanderson, M. R., Yu, D. J., and Sivapalan, M.: Expanding the Scope and Foundation of Sociohydrology as the Science of Coupled Human-Water Systems, Water Resour. Res., 55, 874–887, https://doi.org/10.1029/2018WR024088, 2019.
Koutroulis, A. G., Grillakis, M. G., Tsanis, I. K., and Jacob, D.: Mapping the vulnerability of European summer tourism under 2 °C global warming, Climatic Change, 151, 157–171, https://doi.org/10.1007/s10584-018-2298-8, 2018.
Koutsoyiannis, D.: Hydrology and change, Hydrolog. Sci. J., 58, 1177–1197, https://doi.org/10.1080/02626667.2013.804626, 2013.
Kreibich, H., Van Loon, A. F., Schröter, K., Ward, P. J., Mazzoleni, M., Sairam, N., Abeshu, G. W., Agafonova, S., AghaKouchak, A., Aksoy, H., Alvarez-Garreton, C., Aznar, B., Balkhi, L., Barendrecht, M. H., Biancamaria, S., Bos-Burgering, L., Bradley, C., Budiyono, Y., Buytaert, W., Capewell, L., Carlson, H., Cavus, Y., Couasnon, A., Coxon, G., Daliakopoulos, I., de Ruiter, M. C., Delus, C., Erfurt, M., Esposito, G., François, D., Frappart, F., Freer, J., Frolova, N., Gain, A. K., Grillakis, M., Grima, J. O., Guzmán, D. A., Huning, L. S., Ionita, M., Kharlamov, M., Khoi, D. N., Kieboom, N., Kireeva, M., Koutroulis, A., Lavado-Casimiro, W., Li, H.-Y., LLasat, M. C., Macdonald, D., Mård, J., Mathew-Richards, H., McKenzie, A., Mejia, A., Mendiondo, E. M., Mens, M., Mobini, S., Mohor, G. S., Nagavciuc, V., Ngo-Duc, T., Thao Nguyen Huynh, T., Nhi, P. T. T., Petrucci, O., Nguyen, H. Q., Quintana-Seguí, P., Razavi, S., Ridolfi, E., Riegel, J., Sadik, M. S., Savelli, E., Sazonov, A., Sharma, S., Sörensen, J., Arguello Souza, F. A., Stahl, K., Steinhausen, M., Stoelzle, M., Szalińska, W., Tang, Q., Tian, F., Tokarczyk, T., Tovar, C., Tran, T. V. T., Van Huijgevoort, M. H. J., van Vliet, M. T. H., Vorogushyn, S., Wagener, T., Wang, Y., Wendt, D. E., Wickham, E., Yang, L., Zambrano-Bigiarini, M., Blöschl, G., and Di Baldassarre, G.: The challenge of unprecedented floods and droughts in risk management, Nature, 608, 80–86, https://doi.org/10.1038/s41586-022-04917-5, 2022.
Lesk, C., Rowhani, P., and Ramankutty, N.: Influence of extreme weather disasters on global crop production, Nature, 529, 84–87, https://doi.org/10.1038/nature16467, 2016.
Li, W., Pacheco-Labrador, J., Migliavacca, M., Miralles, D., Hoek van Dijke, A., Reichstein, M., Forkel, M., Zhang, W., Frankenberg, C., Panwar, A., Zhang, Q., Weber, U., Gentine, P., and Orth, R.: Widespread and complex drought effects on vegetation physiology inferred from space, Nat. Commun., 14, 4640, https://doi.org/10.1038/s41467-023-40226-9, 2023.
Lian, X., Piao, S., Li, L. Z. X., Li, Y., Huntingford, C., Ciais, P., Cescatti, A., Janssens, I. A., Peñuelas, J., Buermann, W., Chen, A., Li, X., Myneni, R. B., Wang, X., Wang, Y., Yang, Y., Zeng, Z., Zhang, Y., and McVicar, T. R.: Summer soil drying exacerbated by earlier spring greening of northern vegetation, Sci. Adv., 6, 1–12, https://doi.org/10.1126/sciadv.aax0255, 2020.
Littell, J. S., Peterson, D. L., Riley, K. L., Liu, Y., and Luce, C. H.: A review of the relationships between drought and forest fire in the United States, Global Change Biol., 22, 2353–2369, https://doi.org/10.1111/gcb.13275, 2016.
Liu, T.-M.: The influence of climate change on tourism demand in Taiwan national parks, Tour. Manage. Perspect., 20, 269–275, https://doi.org/10.1016/j.tmp.2016.10.006, 2016.
Lloret, F., Escudero, A., Iriondo, J. M., Martínez-Vilalta, J., and Valladares, F.: Extreme climatic events and vegetation: The role of stabilizing processes, Global Change Biol., 18, 797–805, https://doi.org/10.1111/j.1365-2486.2011.02624.x, 2012.
Luan, X. and Vico, G.: Canopy temperature and heat stress are increased by compound high air temperature and water stress and reduced by irrigation – a modeling analysis, Hydrol. Earth Syst. Sci., 25, 1411–1423, https://doi.org/10.5194/hess-25-1411-2021, 2021.
Lubell, Y., Dondorp, A., Guérin, P. J., Drake, T., Meek, S., Ashley, E., Day, N. P. J., White, N. J., and White, L. J.: Artemisinin resistance – modelling the potential human and economic costs, Malar. J., 13, 452, https://doi.org/10.1186/1475-2875-13-452, 2014.
Luce, C. H., Vose, J. M., Pederson, N., Campbell, J., Millar, C., Kormos, P., and Woods, R.: Contributing factors for drought in United States forest ecosystems under projected future climates and their uncertainty, Forest Ecol. Manage., 380, 299–308, https://doi.org/10.1016/j.foreco.2016.05.020, 2016.
Machado-Silva, F., Peres, L. F., Gouveia, C. M., Enrich-Prast, A., Peixoto, R. B., Pereira, J. M. C., Marotta, H., Fernandes, P. J. F., and Libonati, R.: Drought Resilience Debt Drives NPP Decline in the Amazon Forest, Global Biogeochem. Cy., 35, e2021GB007004, https://doi.org/10.1029/2021GB007004, 2021.
Madadgar, S., AghaKouchak, A., Farahmand, A., and Davis, S. J.: Probabilistic estimates of drought impacts on agricultural production, Geophys. Res. Lett., 44, 7799–7807, https://doi.org/10.1002/2017GL073606, 2017.
Mahecha, M. D., Bastos, A., Bohn, F. J., Eisenhauer, N., Feilhauer, H., Hartmann, H., Hickler, T., Kalesse-Los, H., Migliavacca, M., Otto, F. E. L., Peng, J., Quaas, J., Tegen, I., Weigelt, A., Wendisch, M., and Wirth, C.: Biodiversity loss and climate extremes – study the feedbacks, Nature, 612, 30–32, https://doi.org/10.1038/d41586-022-04152-y, 2022.
Margariti, J., Rangecroft, S., Parry, S., Wendt, D. E., and Van Loon, A. F.: Anthropogenic activities alter drought termination, Elem. Sci. Anthr., 7, 27, https://doi.org/10.1525/elementa.365, 2019.
Marini, L., St-Martin, A., Vico, G., Baldoni, G., Berti, A., Blecharczyk, A., Malecka-Jankowiak, I., Morari, F., Sawinska, Z., and Bommarco, R.: Crop rotations sustain cereal yields under a changing climate, Environ. Res. Lett., 15, 124011, https://doi.org/10.1088/1748-9326/abc651, 2020.
Martínez-Vilalta, J. and Lloret, F.: Drought-induced vegetation shifts in terrestrial ecosystems: The key role of regeneration dynamics, Global Planet. Change, 144, 94–108, https://doi.org/10.1016/j.gloplacha.2016.07.009, 2016.
Matanó, A., Berghuijs, W. R., Mazzoleni, M., de Ruiter, M. C., Ward, P. J., and Van Loon, A. F.: Compound and consecutive drought-flood events at a global scale, Environ. Res. Lett., 19, 64048, https://doi.org/10.1088/1748-9326/ad4b46, 2024.
McCarthy, N., Kilic, T., Brubaker, J., Murray, S., and De La Fuente, A.: Droughts and floods in Malawi: Impacts on crop production and the performance of sustainable land management practices under weather extremes, Environ. Dev. Econ., 26, 432–449, https://doi.org/10.1017/S1355770X20000455, 2021.
McDowell, N. G., Sapes, G., Pivovaroff, A., Adams, H. D., Allen, C. D., Anderegg, W. R. L., Arend, M., Breshears, D. D., Brodribb, T., Choat, B., Cochard, H., De Cáceres, M., De Kauwe, M. G., Grossiord, C., Hammond, W. M., Hartmann, H., Hoch, G., Kahmen, A., Klein, T., Mackay, D. S., Mantova, M., Martínez-Vilalta, J., Medlyn, B. E., Mencuccini, M., Nardini, A., Oliveira, R. S., Sala, A., Tissue, D. T., Torres-Ruiz, J. M., Trowbridge, A. M., Trugman, A. T., Wiley, E., and Xu, C.: Mechanisms of woody-plant mortality under rising drought, CO2 and vapour pressure deficit, Nat. Rev. Earth Environ., 3, 294–308, https://doi.org/10.1038/s43017-022-00272-1, 2022.
McKee, T. B., Nolan, J., and Kleist, J.: The relationship of drought frequency and duration yo time scales, Eighth Conf. Appl. Climatol., 17–22 January 1993, Anaheim, California, 1–6, https://doi.org/10.1002/jso.23002, 1993.
Meddens, A. J. H., Hicke, J. A., and Ferguson, C. A.: Spatiotemporal patterns of observed bark beetle-caused tree mortality in British Columbia and the western United States, Ecol. Appl., 22, 1876–1891, https://doi.org/10.1890/11-1785.1, 2012.
Mendoza, H., Rohse, M., Van Loon, A. F., and Koehler, J.: Disentangling adaptation: From dependent to transformative adaptation, Global Environ. Chang., in review, 2024.
Miralles, D. G., Gentine, P., Seneviratne, S. I., and Teuling, A. J.: Land–atmospheric feedbacks during droughts and heatwaves: state of the science and current challenges, Ann. N.Y. Acad. Sci., 1436, 19–35, https://doi.org/10.1111/nyas.13912, 2019.
Mishra, A. K. and Singh, V. P.: A review of drought concepts, J. Hydrol., 391, 202–216, https://doi.org/10.1016/j.jhydrol.2010.07.012, 2010.
Mishra, A. K. and Singh, V. P.: Drought modeling – A review, J. Hydrol., 403, 157–175, https://doi.org/10.1016/j.jhydrol.2011.03.049, 2011.
Monte, B., Goldenfum, J., Michel, G., and Cavalcanti, J. R.: Terminology of natural hazards and disasters: A review and the case of Brazil, Int. J. Disast. Risk Reduct., 52, 101970, https://doi.org/10.1016/j.ijdrr.2020.101970, 2020.
Mora, C., McKenzie, T., Gaw, I. M., Dean, J. M., von Hammerstein, H., Knudson, T. A., Setter, R. O., Smith, C. Z., Webster, K. M., Patz, J. A., and Franklin, E. C.: Over half of known human pathogenic diseases can be aggravated by climate change, Nat. Clim. Change, 12, 869–875, https://doi.org/10.1038/s41558-022-01426-1, 2022.
Morrison, T. H., Adger, W. N., Brown, K., Lemos, M. C., Huitema, D., Phelps, J., Evans, L., Cohen, P., Song, A. M., Turner, R., Quinn, T., and Hughes, T. P.: The black box of power in polycentric environmental governance, Global Environ. Change, 57, 101934, https://doi.org/10.1016/j.gloenvcha.2019.101934, 2019.
Mu, M., De Kauwe, M. G., Ukkola, A. M., Pitman, A. J., Guo, W., Hobeichi, S., and Briggs, P. R.: Exploring how groundwater buffers the influence of heatwaves on vegetation function during multi-year droughts, Earth Syst. Dynam., 12, 919–938, https://doi.org/10.5194/esd-12-919-2021, 2021.
Mudelsee, M.: Long memory of rivers from spatial aggregation, Water Resour. Res., 43, W01202, https://doi.org/10.1029/2006WR005721, 2007.
Müller, L. M. and Bahn, M.: Drought legacies and ecosystem responses to subsequent drought, Global Change Biol., 28, 5086–5103, https://doi.org/10.1111/gcb.16270, 2022.
Nagavciuc, V., Mursa, A., Ionita, M., Sfeclă, V., Popa, I., and Roibu, C.-C.: An Overview of Extreme Years in Quercus sp. Tree Ring Records from the Northern Moldavian Plateau, Forests, 14, 894, https://doi.org/10.3390/F14050894, 2023.
Nawrotzki, R. J. and DeWaard, J.: Putting trapped populations into place: climate change and inter-district migration flows in Zambia, Reg. Environ. Change, 18, 533–546, https://doi.org/10.1007/s10113-017-1224-3, 2018.
Naylor, A., Ford, J., Pearce, T., and Van Alstine, J.: Conceptualizing Climate Vulnerability in Complex Adaptive Systems, One Earth, 2, 444–454, https://doi.org/10.1016/j.oneear.2020.04.011, 2020.
NDMA: Kenya National Drought Management Authority, Kenya Natl. Drought Manag. Auth., https://ndma.go.ke/about-ndma/ (last access: 21 January 2024), 2023.
Nohrstedt, D.: When do disasters spark transformative policy change and why?, Policy Polit., 50, 425–441, https://doi.org/10.1332/030557321x16508834302815, 2022.
O'Brien, L. V, Berry, H. L., Coleman, C., and Hanigan, I. C.: Drought as a mental health exposure, Environ. Res, 131, 181–187, https://doi.org/10.1016/j.envres.2014.03.014, 2014.
Odongo, R. A., De Moel, H., and Van Loon, A. F.: Propagation from meteorological to hydrological drought in the Horn of Africa using both standardized and threshold-based indices, Nat. Hazards Earth Syst. Sci., 23, 2365–2386, https://doi.org/10.5194/nhess-23-2365-2023, 2023.
Öhrn, P., Berlin, M., Elfstrand, M., Krokene, P., and Jönsson, A. M.: Seasonal variation in Norway spruce response to inoculation with bark beetle-associated bluestain fungi one year after a severe drought, Forest Ecol. Manage., 496, 119443, https://doi.org/10.1016/j.foreco.2021.119443, 2021.
Ojha, C., Shirzaei, M., Werth, S., Argus, D. F., and Farr, T. G.: Sustained Groundwater Loss in California's Central Valley Exacerbated by Intense Drought Periods, Water Resour. Res., 54, 4449–4460, https://doi.org/10.1029/2017WR022250, 2018.
Ostrom, E.: A General Framework for Analyzing Sustainability of Social-Ecological Systems, Science, 325, 419–422, https://doi.org/10.1126/science.1172133, 2009.
Overpeck, J. T. and Udall, B.: Climate change and the aridification of North America, P. Natl. Acad. Sci. USA, 117, 11856–11858, https://doi.org/10.1073/pnas.2006323117, 2020.
Pandey, S. and Bhandari, H.: Drought, coping mechanisms and poverty, Insights from rainfed rice farming in Asia, Rome, Italy, https://www.farm-d.org/app/uploads/2019/05/drought.pdf (last access: 5 July 2024), 2009.
Parry, S., Wilby, L. R., Prudhomme, C., and Wood, J. P.: A systematic assessment of drought termination in the United Kingdom, Hydrol. Earth Syst. Sci., 20, 4265–4281, https://doi.org/10.5194/hess-20-4265-2016, 2016.
Pauloo, R. A., Escriva-Bou, A., Dahlke, H., Fencl, A., Guillon, H., and Fogg, G. E.: Domestic well vulnerability to drought duration and unsustainable groundwater management in California's Central Valley, Environ. Res. Lett., 15, 44010, https://doi.org/10.1088/1748-9326/ab6f10, 2020.
Peixoto, T. P. and Rosvall, M.: Modelling sequences and temporal networks with dynamic community structures, Nat. Commun., 8, 1–12, https://doi.org/10.1038/s41467-017-00148-9, 2017.
Pendergrass, A. G., Meehl, G. A., Pulwarty, R., Hobbins, M., Hoell, A., AghaKouchak, A., Bonfils, C. J. W., Gallant, A. J. E., Hoerling, M., Hoffmann, D., Kaatz, L., Lehner, F., Llewellyn, D., Mote, P., Neale, R. B., Overpeck, J. T., Sheffield, A., Stahl, K., Svoboda, M., Wheeler, M. C., Wood, A. W., and Woodhouse, C. A.: Flash droughts present a new challenge for subseasonal-to-seasonal prediction, Nat. Clim. Change, 10, 191–199, https://doi.org/10.1038/s41558-020-0709-0, 2020.
Peters, W., van der Velde, I. R., van Schaik, E., Miller, J. B., Ciais, P., Duarte, H. F., van der Laan-Luijkx, I. T., van der Molen, M. K., Scholze, M., Schaefer, K., Vidale, P. L., Verhoef, A., Wårlind, D., Zhu, D., Tans, P. P., Vaughn, B., and White, J. W. C.: Increased water-use efficiency and reduced CO2 uptake by plants during droughts at a continental scale, Nat. Geosci., 11, 744–748, https://doi.org/10.1038/s41561-018-0212-7, 2018.
Peterson, T. J., Saft, M., Peel, M. C., and John, A.: Watersheds may not recover from drought, Science, 372, 745–749, https://doi.org/10.1126/science.abd5085, 2021.
Pittelkow, C. M., Linquist, B. A., Lundy, M. E., Liang, X., van Groenigen, K. J., Lee, J., van Gestel, N., Six, J., Venterea, R. T., and van Kessel, C.: When does no-till yield more? A global meta-analysis, Field Crop. Res., 183, 156–168, https://doi.org/10.1016/j.fcr.2015.07.020, 2015.
Placella, S. A., Brodie, E. L., and Firestone, M. K.: Rainfall-induced carbon dioxide pulses result from sequential resuscitation of phylogenetically clustered microbial groups, P. Natl. Acad. Sci. USA, 109, 10931–10936, https://doi.org/10.1073/pnas.1204306109, 2012.
Pongratz, J., Reick, C. H., Raddatz, T., and Claussen, M.: Effects of anthropogenic land cover change on the carbon cycle of the last millennium, Global Biogeochem. Cy., 23, 1–13, https://doi.org/10.1029/2009GB003488, 2009.
Preiser, R., Biggs, R., De Vos, A., and Folke, C.: Social-ecological systems as complex adaptive systems, Ecol. Soc., 23, 46, https://doi.org/10.5751/ES-10558-230446, 2018.
Pribyl, K., Nash, D. J., Klein, J., and Endfield, G. H.: The role of drought in agrarian crisis and social change: the famine of the 1890s in south-eastern Africa, Reg. Environ. Change, 19, 2683–2695, https://doi.org/10.1007/s10113-019-01563-y, 2019.
Pulwarty, R. S. and Sivakumar, M. V: Information systems in a changing climate: Early warnings and drought risk management, Weather Clim. Extrem., 3, 14–21, https://doi.org/10.1016/j.wace.2014.03.005, 2014.
Quandt, A.: The role of qualitative social science. Discussion of “Guiding principles for hydrologists conducting interdisciplinary research and fieldwork with participants”, Hydrolog. Sci. J., 67, 1141–1144, https://doi.org/10.1080/02626667.2022.2060107, 2022.
Raderschall, C. A., Vico, G., Lundin, O., Taylor, A. R., and Bommarco, R.: Water stress and insect herbivory interactively reduce crop yield while the insect pollination benefit is conserved, Global Change Biol., 27, 71–83, https://doi.org/10.1111/gcb.15386, 2021.
Raju, E., Boyd, E., and Otto, F.: Stop blaming the climate for disasters, Commun. Earth Environ., 3, 1, https://doi.org/10.1038/s43247-021-00332-2, 2022.
Rammel, C., Stagl, S., and Wilfing, H.: Managing complex adaptive systems – A co-evolutionary perspective on natural resource management, Ecol. Econ., 63, 9–21, https://doi.org/10.1016/j.ecolecon.2006.12.014, 2007.
Rangecroft, S., Van Loon, A. F., Maureira, H., Verbist, K., and Hannah, D. M.: An observation-based method to quantify the human influence on hydrological drought: upstream–downstream comparison, Hydrolog. Sci. J., 64, 276–287, https://doi.org/10.1080/02626667.2019.1581365, 2019.
Rangecroft, S., Rohse, M., Banks, E. W., Day, R., Di Baldassarre, G., Frommen, T., Hayashi, Y., Höllermann, B., Lebek, K., Mondino, E., Rusca, M., Wens, M., and Van Loon, A. F.: Guiding principles for hydrologists conducting interdisciplinary research and fieldwork with participants, Hydrolog. Sci. J., 66, 214–225, https://doi.org/10.1080/02626667.2020.1852241, 2021.
Ray, D. K., Gerber, J. S., MacDonald, G. K., and West, P. C.: Climate variation explains a third of global crop yield variability, Nat. Commun., 6, 5989, https://doi.org/10.1038/ncomms6989, 2015.
Redman, C. and Kinzig, A.: Resilience of past landscapes: Resilience theory, society, and the Longue Durée, Ecol. Soc., 7, 1–15, 2003.
Reichstein, M., Bahn, M., Ciais, P., Frank, D., Mahecha, M. D., Seneviratne, S. I., Zscheischler, J., Beer, C., Buchmann, N., Frank, D. C., Papale, D., Rammig, A., Smith, P., Thonicke, K., Van Der Velde, M., Vicca, S., Walz, A., and Wattenbach, M.: Climate extremes and the carbon cycle, Nature, 500, 287–295, https://doi.org/10.1038/nature12350, 2013.
Renwick, L. L. R., Deen, W., Silva, L., Gilbert, M. E., Maxwell, T., Bowles, T. M., and Gaudin, A. C. M.: Long-term crop rotation diversification enhances maize drought resistance through soil organic matter, Environ. Res. Lett., 16, 084067, https://doi.org/10.1088/1748-9326/ac1468, 2021.
Ribeiro Neto, G. G., Melsen, L. A., Martins, E. S. P. R. P. R., Walker, D. W., and van Oel, P. R.: Drought Cycle Analysis to Evaluate the Influence of a Dense Network of Small Reservoirs on Drought Evolution, Water Resour. Res., 58, e2021WR030799, https://doi.org/10.1029/2021WR030799, 2022.
Rogosch, J., Tonkin, J., Lytle, D., Merritt, D., Reynolds, L., and Olden, J.: Increasing drought favors nonnative fishes in a dryland river: evidence from a multispecies demographic model, Ecosphere, 10, e02681, https://doi.org/10.1002/ecs2.2681, 2019.
Roobavannan, M., Kandasamy, J., Pande, S., Vigneswaran, S., and Sivapalan, M.: Role of Sectoral Transformation in the Evolution of Water Management Norms in Agricultural Catchments: A Sociohydrologic Modeling Analysis, Water Resour. Res., 53, 8344–8365, https://doi.org/10.1002/2017WR020671, 2017.
Rossi, L., Wens, M., De Moel, H., Cotti, D., Siemons, A. S., Toreti, A., Maetens, W., Masante, D., Van Loon, A., Hagenlocher, M., Rudari, R., Naumann, G., Meroni, M., Avanzi, F., Isabellon, M., and Barbosa, P.: European Drought Risk Atlas, Publ. Off. Eur. Union, https://doi.org/10.2760/608737, 2023.
Ruehr, N. K., Grote, R., Mayr, S., and Arneth, A.: Beyond the extreme: Recovery of carbon and water relations in woody plants following heat and drought stress, Tree Physiol., 39, 1285–1299, https://doi.org/10.1093/treephys/tpz032, 2019.
Ruiz-Pérez, G. and Vico, G.: Effects of Temperature and Water Availability on Northern European Boreal Forests, Front. Forest. Global Change, 3, 1–18, https://doi.org/10.3389/ffgc.2020.00034, 2020.
Sabatier, P.: From Policy Implementation to Policy Change: A Personal Odyssey, in: Reform and Change in Higher Education, edited by: Gornitzka, Å., Kogan, M., and Amaral, A., Higher Education Dynamics, vol. 8, Springer, Dordrecht, https://doi.org/10.1007/1-4020-3411-3_2, ISBN 978-1-4020-3402-2, 2005.
Saft, M., Western, A. W., Zhang, L., Peel, M. C., and Potter, N. J.: The influence of multiyear drought on the annual rainfall-runoff relationship: An Australian perspective, Water Resour. Res., 51, 2444–2463, https://doi.org/10.1002/2014WR015348, 2015.
Salite, D. and Poskitt, S.: Managing the impacts of drought: The role of cultural beliefs in small-scale farmers' responses to drought in Gaza Province, southern Mozambique, Int. J. Disast. Risk Reduct., 41, 101298, https://doi.org/10.1016/j.ijdrr.2019.101298, 2019.
Samuel, S., Dosio, A., Mphale, K., Faka, D. N., and Wiston, M.: Comparison of multi-model ensembles of global and regional climate model projections for daily characteristics of precipitation over four major river basins in southern Africa. Part II: Future changes under 1.5 °C, 2.0 °C and 3.0 °C warming levels, Atmos. Res., 293, 106921, https://doi.org/10.1016/j.atmosres.2023.106921, 2023.
Savelli, E., Rusca, M., Cloke, H., and Di Baldassarre, G.: Don't blame the rain: Social power and the 2015–2017 drought in Cape Town, J. Hydrol., 594, 125953, https://doi.org/10.1016/j.jhydrol.2020.125953, 2021.
Scheffer, M., Carpenter, S., Foley, J. A., Folke, C., and Walker, B.: Catastrophic shifts in ecosystems, Nature, 413, 591–596, https://doi.org/10.1038/35098000, 2001.
Schimel, J. P.: Life in dry soils: Effects of drought on soil microbial communities and processes, Annu. Rev. Ecol. Evol. Syst., 49, 409–432, https://doi.org/10.1146/annurev-ecolsys-110617-062614, 2018.
Schipanski, M. E., Sanderson, M. R., Méndez-Barrientos, L. E., Kremen, A., Gowda, P., Porter, D., Wagner, K., West, C., Rice, C. W., Marsalis, M., Guerrero, B., Haacker, E., Dobrowolski, J., Ray, C., and Auvermann, B.: Moving from measurement to governance of shared groundwater resources, Nat. Water, 1, 30–36, https://doi.org/10.1038/s44221-022-00008-x, 2023.
Schipper, E. L. F.: Maladaptation: When Adaptation to Climate Change Goes Very Wrong, One Earth, 3, 409–414, https://doi.org/10.1016/j.oneear.2020.09.014, 2020.
Schneider, F., Don, A., Hennings, I., Schmittmann, O., and Seidel, S. J.: The effect of deep tillage on crop yield – What do we really know?, Soil Till. Res., 174, 193–204, https://doi.org/10.1016/j.still.2017.07.005, 2017.
Schumacher, D. L., Keune, J., Dirmeyer, P., and Miralles, D. G.: Drought self-propagation in drylands due to land–atmosphere feedbacks, Nat. Geosci., 15, 262–268, https://doi.org/10.1038/s41561-022-00912-7, 2022.
Schwalm, C. R., Anderegg, W. R. L., Michalak, A. M., Fisher, J. B., Biondi, F., Koch, G., Litvak, M., Ogle, K., Shaw, J. D., Wolf, A., Huntzinger, D. N., Schaefer, K., Cook, R., Wei, Y., Fang, Y., Hayes, D., Huang, M., Jain, A., and Tian, H.: Global patterns of drought recovery, Nature, 548, 202–205, https://doi.org/10.1038/nature23021, 2017.
Seager, R., Osborn, T. J., Kushnir, Y., Simpson, I. R., Nakamura, J., and Liu, H.: Climate variability and change of mediterranean-type climates, J. Climate, 32, 2887–2915, https://doi.org/10.1175/JCLI-D-18-0472.1, 2019.
Sears, R. R., Shah, A. N., Lehmann, L. M., and Ghaley, B. B.: Comparison of resilience of different plant teams to drought and temperature extremes in Denmark in sole and intercropping systems, Acta Agric. Scand. Sect. B, 71, 645–655, https://doi.org/10.1080/09064710.2021.1936621, 2021.
Sena, A., Ebi, K. L., Freitas, C., Corvalan, C., and Barcellos, C.: Indicators to measure risk of disaster associated with drought: Implications for the health sector, PLoS One, 12, e0181394, https://doi.org/10.1371/journal.pone.0181394, 2017.
Shah, J., Hari, V., Rakovec, O., Markonis, Y., Samaniego, L., Mishra, V., Hanel, M., Hinz, C., and Kumar, R.: Increasing footprint of climate warming on flash droughts occurrence in Europe, Environ. Res. Lett., 17, 064017, https://doi.org/10.1088/1748-9326/ac6888, 2022.
Shaked, H. and Schechter, C.: Systems Thinking for School Leaders Holistic Leadership for Excellence in Education, in: 1st Edn., Springer, Cham, Switzerland, ISBN 978-3-319-53570-8, https://doi.org/10.1007/978-3-319-53571-5, 2017.
Shepherd, T. G., Boyd, E., Calel, R. A., Chapman, S. C., Dessai, S., Dima-West, I. M., Fowler, H. J., James, R., Maraun, D., Martius, O., Senior, C. A., Sobel, A. H., Stainforth, D. A., Tett, S. F. B., Trenberth, K. E., van den Hurk, B. J. J. M., Watkins, N. W., Wilby, R. L., and Zenghelis, D. A.: Storylines: an alternative approach to representing uncertainty in physical aspects of climate change, Climatic Change, 151, 555–571, https://doi.org/10.1007/s10584-018-2317-9, 2018.
Shiferaw, B., Tesfaye, K., Kassie, M., Abate, T., Prasanna, B. M., and Menkir, A.: Managing vulnerability to drought and enhancing livelihood resilience in sub-Saharan Africa: Technological, institutional and policy options, Weather Clim. Extrem., 3, 67–79, https://doi.org/10.1016/j.wace.2014.04.004, 2014.
Shyrokaya, A., Pappenberger, F., Pechlivanidis, I., Messori, G., Khatami, S., Mazzoleni, M., and Di Baldassarre, G.: Advances and gaps in the science and practice of impact-based forecasting of droughts, WIREs Water, 1, e1698, https://doi.org/10.1002/wat2.1698, 2023.
Shyrokaya, A., Messori, G., Pechlivanidis, I., Pappenberger, F., Cloke, H. L., and Di Baldassarre, G.: Significant relationships between drought indicators and impacts for the 2018–2019 drought in Germany, Environ. Res. Lett., 19, 014037, https://doi.org/10.1088/1748-9326/ad10d9, 2024.
Siirila-Woodburn, E. R., Rhoades, A. M., Hatchett, B. J., Huning, L. S., Szinai, J., Tague, C., Nico, P. S., Feldman, D. R., Jones, A. D., Collins, W. D., and Kaatz, L.: A low-to-no snow future and its impacts on water resources in the western United States, Nat. Rev. Earth Environ., 2, 800–819, https://doi.org/10.1038/s43017-021-00219-y, 2021.
Sillmann, J., Christensen, I., Hochrainer-Stigler, S., Huang-Lachmann, J., Juhola, S., Kornhuber, K., Mahecha, M., Mechler, R., Reichstein, M., Ruane, A. C., Schweizer, P.-J., and Williams, S.: Briefing note on systemic risk, Paris, France, https://www.undrr.org/media/78607/download?startDownload=20240905 (last access: 5 July 2024), 2022.
Simpson, N. P., Shearing, C. D., and Dupont, B.: Climate gating: A case study of emerging responses to Anthropocene Risks, Clim. Risk Manage., 26, 100196, https://doi.org/10.1016/j.crm.2019.100196, 2019.
SISSA: Latin American drought forecast, Drought Inf. Syst. South. South Am., https://sissa.crc-sas.org/ (last access: 21 January 2024), 2023.
Sivakumar, M. V., Stefanski, R., Bazza, M., Zelaya, S., Magalhaes, D., and Wilhite, A. R.: High Level Meeting on National Drought Policy: Summary and major outcomes, Weather Clim. Extrem., 3, 126–132, 2014.
Sivapalan, M., Savenije, H. H. G., and Blöschl, G.: Socio-hydrology A new science of people and water, Hydrol. Process., 26, 1270–1276, 2012.
Slette, I. J., Post, A. K., Awad, M., Even, T., Punzalan, A., Williams, S., Smith, M. D., and Knapp, A. K.: How ecologists define drought, and why we should do better, Global Change Biol., 25, 3193–3200, https://doi.org/10.1111/gcb.14747, 2019.
Smith, R. G., Knight, R., Chen, J., Reeves, J. A., Zebker, H. A., Farr, T., and Liu, Z.: Estimating the permanent loss of groundwater storage in the southern San Joaquin Valley, California, Water Resour. Res., 53, 2133–2148, https://doi.org/10.1002/2016WR019861, 2017.
Sousa, C., Teixeira, L., and Fouto, N.: Midterm impacts of a water drought experience: evaluation of consumption changes in São Paulo, Brazil, Water Policy, 24, 179–191, https://doi.org/10.2166/wp.2021.120, 2022.
Srinivasan, V., Lambin, E. F., Gorelick, S. M., Thompson, B. H., and Rozelle, S.: The nature and causes of the global water crisis: Syndromes from a meta-analysis of coupled human-water studies, Water Resour. Res., 48, 1–16, https://doi.org/10.1029/2011WR011087, 2012.
Stahl, K., Kohn, I., Blauhut, V., Urquijo, J., De Stefano, L., Acácio, V., Dias, S., Stagge, J. H., Tallaksen, L. M., Kampragou, E., Van Loon, A. F., Barker, L. J., Melsen, L. A., Bifulco, C., Musolino, D., De Carli, A., Massarutto, A., Assimacopoulos, D., and Van Lanen, H. A. J.: Impacts of European drought events: Insights from an international database of text-based reports, Nat. Hazards Earth Syst. Sci., 16, 801–819, https://doi.org/10.5194/nhess-16-801-2016, 2016.
Steel, Z. L., Jones, G. M., Collins, B. M., Green, R., Koltunov, A., Purcell, K. L., Sawyer, S. C., Slaton, M. R., Stephens, S. L., Stine, P., and Thompson, C.: Mega-disturbances cause rapid decline of mature conifer forest habitat in California, Ecol. Appl., 33, e2763, https://doi.org/10.1002/eap.2763, 2023.
Steffen, W., Richardson, K., Rockström, J., Schellnhuber, H. J., Dube, O. P., Dutreuil, S., Lenton, T. M., and Lubchenco, J.: The emergence and evolution of Earth System Science, Nat. Rev. Earth Environ., 1, 54–63, https://doi.org/10.1038/s43017-019-0005-6, 2020.
Stephens, S. L., Collins, B. M., Fettig, C. J., Finney, M. A., Hoffman, C. M., Knapp, E. E., North, M. P., Safford, H., and Wayman, R. B.: Drought, Tree Mortality, and Wildfire in Forests Adapted to Frequent Fire, Bioscience, 68, 77–88, https://doi.org/10.1093/biosci/bix146, 2018.
Stocker, B. D., Zscheischler, J., Keenan, T. F., Prentice, I. C., Seneviratne, S. I., and Peñuelas, J.: Drought impacts on terrestrial primary production underestimated by satellite monitoring, Nat. Geosci., 12, 264–270, https://doi.org/10.1038/s41561-019-0318-6, 2019.
Stoelzle, M., Staudinger, M., Stahl, K., and Weiler, M.: Stress testing as complement to climate scenarios: recharge scenarios to quantify streamflow drought sensitivity, Proc. IAHS, 383, 43–50, https://doi.org/10.5194/piahs-383-43-2020, 2020.
Strang, V.: Integrating The Social and Natural Sciences in Environmental Research: A Discussion Paper, Environ. Dev. Sustainabil., 11, 1–18, https://doi.org/10.1007/s10668-007-9095-2, 2009.
Suarez, M. L. and Kitzberger, T.: Recruitment patterns following a severe drought: long-term compositional shifts in Patagonian forests, Can. J. Forest. Res., 38, 3002–3010, https://doi.org/10.1139/X08-149, 2008.
Sungmin, O. and Park, S. K.: Global ecosystem responses to flash droughts are modulated by background climate and vegetation conditions, Commun. Earth Environ., 5, 88, https://doi.org/10.1038/s43247-024-01247-4, 2024.
Sutanto, S. J. and Van Lanen, H. A. J.: Catchment memory explains hydrological drought forecast performance, Sci. Rep., 12, 2689, https://doi.org/10.1038/s41598-022-06553-5, 2022.
Sutanto, S. J., Wetterhall, F., and Van Lanen, H. A. J.: Hydrological drought forecasts outperform meteorological drought forecasts, Environ. Res. Lett., 15, 084010, https://doi.org/10.1088/1748-9326/ab8b13, 2020.
Svoboda, M. and Fuchs, B.: Handbook of Drought Indicators and Indices, World Meteorological Organization (WMO) and Global Water Partnership (GWP), Integrated Drought Management Programme (IDMP), Integrated Drought Management Tools and Guidelines Series 2, Geneva, ISBN 978-92-63-11173-9, https://www.droughtmanagement.info/literature/GWP_Handbook_of_Drought_Indicators_and_Indices_2016.pdf (last access: 5 July 2024), 2016.
Tallaksen, L. M. and Stahl, K.: Spatial and temporal patterns of large-scale droughts in Europe Model dispersion and performance, Geophys. Res. Lett., 41, 429–434, https://doi.org/10.1002/2013GL058573, 2014.
Taylor, J. G., Stewart, T. R., and Downton, M.: Perceptions of drought in the Ogallala Aquifer region, Environ. Behav., 20, 150–175, 1988.
Teisman, G. and Edelenbos, J.: Towards a Perspective of System Synchronization in Water Governance: A Synthesis of Empirical Lessons and Complexity Theories, Int. Rev. Adm. Sci., 77, 101–118, https://doi.org/10.1177/0020852310390121, 2011.
Tellman, B., Bausch, J. C., Eakin, H., Anderies, J. M., Mazari-Hiriart, M., Manuel-Navarrete, D., and Redman, C. L.: Adaptive pathways and coupled infrastructure: Seven centuries of adaptation to water risk and the production of vulnerability in Mexico city, Ecol. Soc., 23, 1–20, https://doi.org/10.5751/ES-09712-230101, 2018.
Teutschbein, C., Quesada Montano, B., Todorović, A., and Grabs, T.: Streamflow droughts in Sweden: Spatiotemporal patterns emerging from six decades of observations, J. Hydrol.: Reg. Stud., 42, 101171, https://doi.org/10.1016/j.ejrh.2022.101171, 2022.
Teutschbein, C., Albrecht, F., Blicharska, M., Tootoonchi, F., Stenfors, E., and Grabs, T.: Drought hazards and stakeholder perception: Unraveling the interlinkages between drought severity, perceived impacts, preparedness, and management, Ambio, 52, 1262–1281, https://doi.org/10.1007/s13280-023-01849-w, 2023.
Tijdeman, E., Blauhut, V., Stoelzle, M., Menzel, L., and Stahl, K.: Different drought types and the spatial variability in their hazard, impact, and propagation characteristics, Nat. Hazards Earth Syst. Sci., 22, 2099–2116, https://doi.org/10.5194/nhess-22-2099-2022, 2022.
Tombesi, S., Nardini, A., Frioni, T., Soccolini, M., Zadra, C., Farinelli, D., Poni, S., and Palliotti, A.: Stomatal closure is induced by hydraulic signals and maintained by ABA in drought-stressed grapevine, Sci. Rep., 5, 12449, https://doi.org/10.1038/srep12449, 2015.
Treibich, C., Bell, E., Lépine, A., and Blanc, E.: From a drought to HIV: An analysis of the effect of droughts on transactional sex and sexually transmitted infections in Malawi, SSM – Popul. Heal., 19, 101221, https://doi.org/10.1016/j.ssmph.2022.101221, 2022.
Troch, P. A., Carrillo, G., Sivapalan, M., Wagener, T., and Sawicz, K.: Climate-vegetation-soil interactions and long-term hydrologic partitioning: Signatures of catchment co-evolution, Hydrol. Earth Syst. Sci., 17, 2209–2217, https://doi.org/10.5194/hess-17-2209-2013, 2013.
Tversky, A. and Kahneman, D.: Availability: A heuristic for judging frequency and probability, Cognit. Psychol., 5, 207–232, https://doi.org/10.1016/0010-0285(73)90033-9, 1973.
Tyree, M. T. and Ewers, F. W.: The hydraulic architecture of trees and other woody plants, New Phytol., 119, 345–360, https://doi.org/10.1111/j.1469-8137.1991.tb00035.x, 1991.
Ulibarri, N. and Scott, T. A.: Environmental hazards, rigid institutions, and transformative change: How drought affects the consideration of water and climate impacts in infrastructure management, Global Environ. Change, 59, 102005, https://doi.org/10.1016/j.gloenvcha.2019.102005, 2019.
UNCCD: United Nations Convention to Combat Desertification, United Nations Conv. to Combat Desertif., https://www.unccd.int/ (last access: 21 January 2024), 2023.
UNDDR: GAR Special Report on Drought 2021, United Nations Off. Disaster Risk Reduct., https://www.undrr.org/publication/gar-special-report-drought-2021 (last access: 18 January 2024), 2021.
Urquijo-Reguera, J., Gómez-Villarino, M. T., Pereira, D., and De Stefano, L.: An Assessment Framework to Analyze Drought Management Plans: The Case of Spain, Agronomy, 12, 970, https://doi.org/10.3390/agronomy12040970, 2022.
USDM: The U.S. Drought Monitor, Natl. Drought Mitig. Cent., https://droughtmonitor.unl.edu/ (last access: 21 January 2024), 2023.
van der Velde, Y., de Rooij, G. H., and Torfs, P. J. J. F.: Catchment-scale non-linear groundwater-surface water interactions in densely drained lowland catchments, Hydrol. Earth Syst. Sci., 13, 1867–1885, https://doi.org/10.5194/hess-13-1867-2009, 2009.
van der Wiel, K., Lenderink, G., and de Vries, H.: Physical storylines of future European drought events like 2018 based on ensemble climate modelling, Weather Clim. Extrem., 33, 100350, https://doi.org/10.1016/j.wace.2021.100350, 2021.
van Dijk, A. I. J. M., Beck, H. E., Crosbie, R. S., de Jeu, R. A. M., Liu, Y. Y., Podger, G. M., Timbal, B., and Viney, N. R.: The Millennium Drought in southeast Australia (2001–2009): Natural and human causes and implications for water resources, ecosystems, economy, and society, Water Resour. Res., 49, 1040–1057, https://doi.org/10.1002/wrcr.20123, 2013.
van Duinen, R., Filatova, T., Geurts, P., and van der Veen, A.: Empirical analysis of farmers' drought risk perception: Objective factors, personal circumstances, and social influence, Risk Anal., 35, 741–755, 2015.
Vanelli, F. M., Kobiyama, M., and De Brito, M. M.: To which extent are socio-hydrology studies truly integrative? the case of natural hazards and disaster research, Hydrol. Earth Syst. Sci., 26, 2301–2317, https://doi.org/10.5194/hess-26-2301-2022, 2022.
Van Lanen, H. A. J., Laaha, G., Kingston, D. G., Gauster, T., Ionita, M., Vidal, J. P., Vlnas, R., Tallaksen, L. M., Stahl, K., Hannaford, J., Delus, C., Fendekova, M., Mediero, L., Prudhomme, C., Rets, E., Romanowicz, R. J., Gailliez, S., Wong, W. K., Adler, M. J., Blauhut, V., Caillouet, L., Chelcea, S., Frolova, N., Gudmundsson, L., Hanel, M., Haslinger, K., Kireeva, M., Osuch, M., Sauquet, E., Stagge, J. H., and Van Loon, A. F.: Hydrology needed to manage droughts: the 2015 European case, Hydrol. Process., 30, 3097–3104, https://doi.org/10.1002/hyp.10838, 2016.
Van Loon, A. F.: Hydrological drought explained, WIREs Water, 2, 359–392, https://doi.org/10.1002/wat2.1085, 2015.
Van Loon, A. F., Stahl, K., Di Baldassarre, G., Clark, J., Rangecroft, S., Wanders, N., Gleeson, T., Van Dijk, A. I. J. M., Tallaksen, L. M., Hannaford, J., Uijlenhoet, R., Teuling, A. J., Hannah, D. M., Sheffield, J., Svoboda, M., Verbeiren, B., Wagener, T., and Van Lanen, H. A. J.: Drought in a human-modified world: Reframing drought definitions, understanding, and analysis approaches, Hydrol. Earth Syst. Sci., 20, 3631–3650, https://doi.org/10.5194/hess-20-3631-2016, 2016.
Van Loon, A. F., Kumar, R., and Mishra, V.: Testing the use of standardised indices and GRACE satellite data to estimate the European 2015 groundwater drought in near-real time, Hydrol. Earth Syst. Sci., 21, 1947–1971, https://doi.org/10.5194/hess-21-1947-2017, 2017.
Van Loon, A. F., Rangecroft, S., Coxon, G., Werner, M., Wanders, N., Di Baldassarre, G., Tijdeman, E., Bosman, M., Gleeson, T., Nauditt, A., Aghakouchak, A., Breña-Naranjo, J. A., Cenobio-Cruz, O., Costa, A. C., Fendekova, M., Jewitt, G., Kingston, D. G., Loft, J., Mager, S. M., Mallakpour, I., Masih, I., Maureira-Cortés, H., Toth, E., Van Oel, P., Van Ogtrop, F., Verbist, K., Vidal, J.-P., Wen, L., Yu, M., Yuan, X., Zhang, M., and Van Lanen, H. A. J.: Streamflow droughts aggravated by human activities despite management, Environ. Res. Lett., 17, 44059, https://doi.org/10.1088/1748-9326/ac5def, 2022.
van Oel, P. R., Martins, E. S. P. R., Costa, A. C., Wanders, N., and van Lanen, H. A. J.: Diagnosing drought using the downstreamness concept: the effect of reservoir networks on drought evolution, Hydrolog. Sci. J., 63, 979–990, https://doi.org/10.1080/02626667.2018.1470632, 2018.
van Tiel, M., Weiler, M., Freudiger, D., Moretti, G., Kohn, I., Gerlinger, K., and Stahl, K.: Melting Alpine Water Towers Aggravate Downstream Low Flows: A Stress-Test Storyline Approach, Earth's Future, 11, e2022EF003408, https://doi.org/10.1029/2022EF003408, 2023.
Vicente-Serrano, S. M., Beguería, S., and López-Moreno, J. I.: A multiscalar drought index sensitive to global warming: The standardized precipitation evapotranspiration index, J. Climate, 23, 1696–1718, https://doi.org/10.1175/2009JCLI2909.1, 2010.
Vinke, K., Bergmann, J., Blocher, J., Upadhyay, H., and Hoffmann, R.: Migration as Adaptation?, Migrat. Stud., 8, 626–634, https://doi.org/10.1093/migration/mnaa029, 2020.
Vins, H., Bell, J., Saha, S., and Hess, J. J.: The Mental Health Outcomes of Drought: A Systematic Review and Causal Process Diagram, Int. J. Environ. Res. Publ. Health, 12, 13251–13275, https://doi.org/10.3390/ijerph121013251, 2015.
Vogel, C. and Olivier, D.: Re-imagining the potential of effective drought responses in South Africa, Reg. Environ. Change, 19, 1561–1570, https://doi.org/10.1007/s10113-018-1389-4, 2019.
Walker, D. W., Oliveira, J. L., Cavalcante, L., Kchouk, S., Neto Ribeiro, G., Melsen, L. A., Fernandes, F. B. P., Mitroi, V., Gondim, R. S., Martins, E. S. P. R., and van Oel, P.: It's not all about drought: what “drought impacts” monitoring can reveal, Int. J. Disast. Risk Reduct., 103, 104338, https://doi.org/10.1016/j.ijdrr.2024.104338, 2024.
Weiss, H. and Bradley, R. S.: What Drives Societal Collapse?, Science, 291, 609–610, https://doi.org/10.1126/science.1058775, 2001.
Wells, N., Goddard, S., and Hayes, M.: A Self-Calibrating Palmer Drought Severity Index, J. Climate, 17, 2335–2351, https://doi.org/10.1175/1520-0442(2004)017<2335:ASPDSI>2.0.CO;2, 2004.
Wens, M., Johnson, J. M., Zagaria, C., and Veldkamp, T. I. E.: Integrating human behavior dynamics into drought risk assessment A sociohydrologic agent-based, WIREs Water, 6, e1345, https://doi.org/10.1002/wat2.1345, 2019.
Wesselink, A., Kooy, M., and Warner, J.: Sology and hydrosocial analysis toward dialogues across disciplines.pdf, Wiley Interdisciplin. Rev.: Water, 4, e1196, https://doi.org/10.1002/wat2.1196, 2017.
Westra, S. and Zscheischler, J.: Accounting for systemic complexity in the assessment of climate risk, One Earth, 6, 645–655, https://doi.org/10.1016/j.oneear.2023.05.005, 2023.
Wilhite, D. A.: Breaking the hydro-illogical cycle: Progress or status quo for drought management in the United States, Eur. Water, 34, 5–18, 2011.
Wilhite, D. A.: Drought management and policy: Changing the paradigm from crisis to risk management, Eur. Water, 60, 181–187, 2017.
Wilhite, D. A. and Glantz, M. H.: Understanding: the drought phenomenon: the role of definitions, Water Int., 10, 111–120, 1985.
Wilhite, D. A. and Pulwarty, R. S.: National Drought Management Policy Guidelines: A Template for Action, Integr. Drought Manag. Program. Tools Guidel. Ser. 1, 48 pp., ISBN 978-92-63-11164-7, https://www.droughtmanagement.info/literature/IDMP_NDMPG_en.pdf (last access: 5 July 2024) 2014.
Wilhite, D. A., And, M. V. K. S., and Wood, D. A.: Early Warning Systems for Drought Preparedness and Drought Management, World Meteorological Organization, Geneva, http://www.droughtmanagement.info/literature/WMO_early_warning_systems_drought_prepardness_2000.pdf#page=105 (last access: 5 July 2024), 2000.
Williams, A. and de Vries, F. T.: Plant root exudation under drought: implications for ecosystem functioning, New Phytol., 225, 1899–1905, https://doi.org/10.1111/nph.16223, 2020.
Wlostowski, A. N., Jennings, K. S., Bash, R. E., Burkhardt, J., Wobus, C. W., and Aggett, G.: Dry landscapes and parched economies: A review of how drought impacts nonagricultural socioeconomic sectors in the US Intermountain West, WIREs Water, 9, e1571, https://doi.org/10.1002/wat2.1571, 2022.
WMO: Commission on Agricultural Meteorology (CAgM) – Expert Team 3.1 – Report on Drought (2014–2018), Geneva, https://library.wmo.int/idurl/4/57781 (last access: 5 July 2024), 2021.
Wright, A. J., Mommer, L., Barry, K., and van Ruijven, J.: Stress gradients and biodiversity: monoculture vulnerability drives stronger biodiversity effects during drought years, Ecology, 102, 1–10, https://doi.org/10.1002/ecy.3193, 2021.
Wu, M., Schurgers, G., Ahlström, A., Rummukainen, M., Miller, P. A., Smith, B., and May, W.: Impacts of land use on climate and ecosystem productivity over the Amazon and the South American continent, Environ. Res. Lett., 12, 054016, https://doi.org/10.1088/1748-9326/aa6fd6, 2017.
Wu, M., Vico, G., Manzoni, S., Cai, Z., Bassiouni, M., Tian, F., Zhang, J., Ye, K., and Messori, G.: Early Growing Season Anomalies in Vegetation Activity Determine the Large-Scale Climate-Vegetation Coupling in Europe, J. Geophys. Res.-Biogeo., 126, e2020JG006167, https://doi.org/10.1029/2020JG006167, 2021.
Wu, M., Manzoni, S., Vico, G., Bastos, A., de Vries, F. T., and Messori, G.: Drought Legacy in Sub-Seasonal Vegetation State and Sensitivity to Climate Over the Northern Hemisphere, Geophys. Res. Lett., 49, e2022GL098700, https://doi.org/10.1029/2022GL098700, 2022.
Yang, Y., Guan, H., Batelaan, O., McVicar, T. R., Long, D., Piao, S., Liang, W., Liu, B., Jin, Z., and Simmons, C. T.: Contrasting responses of water use efficiency to drought across global terrestrial ecosystems, Sci. Rep., 6, 23284, https://doi.org/10.1038/srep23284, 2016.
Yu, X., Orth, R., Reichstein, M., Bahn, M., Klosterhalfen, A., Knohl, A., Koebsch, F., Migliavacca, M., Mund, M., Nelson, J. A., Stocker, B. D., Walther, S., and Bastos, A.: Contrasting drought legacy effects on gross primary productivity in a mixed versus pure beech forest, Biogeosciences, 19, 4315–4329, https://doi.org/10.5194/bg-19-4315-2022, 2022.
Zaveri, E. D., Damania, R., Engle, N., and Bank, W.: Droughts and Deficits: The Global Impact of Droughts on Economic Growth, World Bank, Washington, D.C., https://www.worldbank.org/en/news/immersive-story/2023/09/12/droughts-and-deficits-the-global-impacts (last access: 5 July 2024), 2023.
Zhang, Q., Ficklin, D. L., Manzoni, S., Wang, L., Way, D., Phillips, R. P., and Novick, K. A.: Response of ecosystem intrinsic water use efficiency and gross primary productivity to rising vapor pressure deficit, Environ. Res. Lett., 14, 074023, https://doi.org/10.1088/1748-9326/ab2603, 2019.
Zhang, T., Niinemets, Ü., Sheffield, J., and Lichstein, J. W.: Shifts in tree functional composition amplify the response of forest biomass to climate, Nature, 556, 99–102, https://doi.org/10.1038/nature26152, 2018.
Zipper, S. C., Qiu, J., and Kucharik, C. J.: Drought effects on US maize and soybean production: Spatiotemporal patterns and historical changes, Environ. Res. Lett., 11, 094021, https://doi.org/10.1088/1748-9326/11/9/094021, 2016.
- Abstract
- Introduction
- Systems theory and drought memory
- Drought as a continuum in different systems
- Drought as a continuum in the system of systems
- Outlook
- Data availability
- Author contributions
- Competing interests
- Disclaimer
- Special issue statement
- Acknowledgements
- Financial support
- Review statement
- References
- Supplement
- Abstract
- Introduction
- Systems theory and drought memory
- Drought as a continuum in different systems
- Drought as a continuum in the system of systems
- Outlook
- Data availability
- Author contributions
- Competing interests
- Disclaimer
- Special issue statement
- Acknowledgements
- Financial support
- Review statement
- References
- Supplement