the Creative Commons Attribution 4.0 License.
the Creative Commons Attribution 4.0 License.
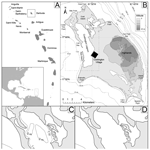
Hurricane Irma: an unprecedented event over the last 3700 years? Geomorphological changes and sedimentological record in Codrington Lagoon, Barbuda
Maude Biguenet
Eric Chaumillon
Pierre Sabatier
Antoine Bastien
Emeline Geba
Fabien Arnaud
Thibault Coulombier
Nathalie Feuillet
Low-lying coasts and small islands, such as in the Lesser Antilles, are particularly vulnerable to hurricane-induced marine floods. In September 2017, category 5 Hurricane Irma, with winds up to 360 km h−1, hit the northern Caribbean islands and caused the destruction of 95 % of the structures on Barbuda Island. We investigated the geomorphological impacts and the sedimentological record related to the storm surge of this hurricane in Barbuda's Codrington Lagoon. Following Hurricane Irma, two wide inlets developed across the Codrington sandy barrier. One of these inlets was enlarged and was still open 4 years later. From available data, it seems that this barrier remained continuous for the last 250 years before Hurricane Irma. At a longer timescale, very high-resolution seismic exploration combined with sediment cores sampled in Codrington Lagoon were used to investigate Irma deposits and environmental changes for the last 3700 years. The evolution from a low-energy small and shallow lagoon to the modern wide and high-energy lagoon recorded by the lagoon sediment fill was related to both long-term sea level rise and subsidence. The top of the lagoon fill consists of a thick and extensive sand sheet recording an abrupt increase in energy. Given its location at the top of the cores and its very recent age, supported by short-lived radionuclide data, together with large inlets opening and barrier erosion after Irma that imply a large sand supply to the lagoon, this sand sheet was attributed to Hurricane Irma. From our cores, it appears that this deposit is unique over more than 3700 years. Both the opening of a new inlet and the thick upper sand sheet support the exceptional character of Irma at the scale of centuries to millennia. Our study reinforces the idea that Hurricane Irma was exceptional in terms of intensity and may be associated with global warming.
- Article
(11706 KB) - Full-text XML
-
Supplement
(68464 KB) - BibTeX
- EndNote
Hurricanes represent a direct threat to coastal territories and populations. Indeed, they generate wind exposure, spin-up tornados, tropical rainfall, storm surge and wind waves. These hurricanes may cause widespread power outages, coastal and inland flooding, beach erosion, infrastructure and structural damage, and direct human deaths (e.g., Gerritsen, 2005; Griffis, 2007; Karim and Mimura, 2008; Breilh et al., 2013; Takagi et al., 2017; Bacopoulos, 2019). Many studies have reported a notable increase in hurricane intensity and associated damage over recent decades (e.g., Kossin et al., 2013, 2020; Holland and Bruyère, 2014; Knutson et al., 2019). Pfleiderer et al. (2022) recently showed that the increase in Atlantic tropical cyclone activity since the 1980s can be robustly ascribed to variations in atmospheric circulation, as well as sea surface temperature (SST) rise. They found that the forced warming trend in Atlantic SSTs over the 1982–2020 period doubled the probability of extremely active tropical cyclone seasons. Given the projected increases in SSTs with increasing warming, this suggests that the probability of extreme seasons (with category 4–5 hurricanes) might be more regular in the future. These high-category hurricanes generate very high-intensity winds that can allow the development of strong surges leading to large coastal flooding. Thus, low-lying coasts and islands are particularly vulnerable to hurricane-induced inundation. Consequently, the sea level rise associated with the increase in hurricane intensity exposes a growing number of them to marine submersion (Bhatia et al., 2019; Pfleiderer et al., 2022). As a result, the local communities will certainly experience another impactful hurricane at some point. The main questions are when and to which extent. Thus, it is crucial to understand the coastline geomorphological and sedimentological changes in response to hurricane processes to better inform and prepare coastal communities (Spiske et al., 2021).
On 30 August 2017, a tropical storm developed west of the Cape Verde Islands (Cangialosi et al., 2018). It was generated by the combination of warm ocean surface temperatures (30–32 ∘C) and little atmospheric shear (Bacopoulos, 2019). The tropical storm transitioned to a category 5 hurricane on 5 September and was named Irma. Hurricane Irma broke meteorological-based records for tropical cyclones in the Atlantic basin (Cangialosi et al., 2018). It was the strongest hurricane to form in the Atlantic Ocean in historical times, outside of the Gulf of Mexico and the Caribbean Sea (Cangialosi et al., 2018). It remained a category 5 hurricane for 3 d, which is the longest category 5 hurricane since satellite storm tracking began (Bacopoulos, 2019). Furthermore, hurricane winds were maintained for a total of 37 h at 185 mph (298 km h−1), which is the longest period of such intensity (maximum sustained winds) among all storms on record (Bacopoulos, 2019). Irma made seven landfalls through the following days, four of which occurred as a category 5 hurricane across the northern Caribbean islands (Barbuda, Saint Martin and Virgin Gorda in the British Virgin Islands and Cayo Romano in Cuba) (Cangialosi et al., 2018). Hurricane Irma brought many coastal hazards such as wind exposure, wind waves, storm surge and tropical rainfall. The consequences included widespread power outages, coastal and inland flooding, beach erosion, infrastructure and other structural damage, and 47 direct human deaths (Bacopoulos, 2019). The damage has cost record-breaking totals, with the amount reaching approximately USD 51 billion for the USA (Bacopoulos, 2019) and USD 3.17 billion for the Lesser Antilles, the Turks and Caicos Islands, and Cuba. The costs reached USD 150 to 300 million for Barbuda Island alone (Cangialosi et al., 2018). The storm's eye passed directly through Barbuda Island, exposing the island at its peak intensity to a mean wind speed of 155 kn (287 km h−1), maximum recorded gusts of wind speed of 360 km h−1 and a minimum pressure of 914 mb for more than 3 h. Irma's catastrophic winds caused destruction across the island, damaging or destroying approximately 95 % of the structures and directly causing the deaths of three people (Cangialosi et al., 2018). The damage to housing and infrastructure was so extensive that all 1800 residents of the island were immediately evacuated post-hurricane to the neighboring island of Antigua (Boger et al., 2020). This decision was made due to the threat posed by category 4 Hurricane Jose, which passed 60 km north of the island on 8 September (Berg, 2018).
West of Barbuda, Codrington Lagoon and its barrier were strongly hit by Irma and have the potential to record older hurricanes. Thus, they are a key area for showing the impacts of such an extreme event. This article aims at documenting the impact of Hurricane Irma on the geomorphology and sedimentation of Codrington Lagoon and barrier. The geomorphological changes in Codrington Lagoon sandy barrier are examined through recent satellite images, as well as seismic profiles, and compared with older satellite images and nautical maps, allowing a 250-year-long observation of the barrier morphology. Additionally, we sampled six sedimentary cores 7 months after the event to document the sedimentary record of this extreme event. Furthermore, the study of the complete sedimentary sequences through a multiproxy approach (sedimentological, geochemical and microfaunal analyses) allowed us to place this event within a long-term perspective and question its relative importance over the last thousands of years.
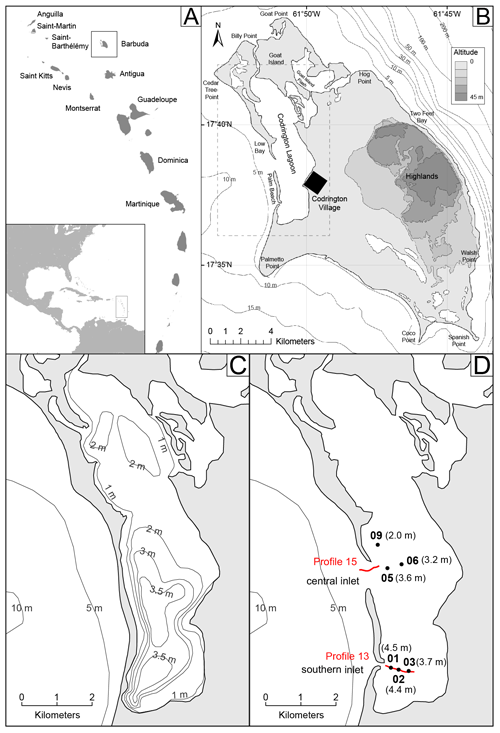
Figure 1(a) The Caribbean Sea and Lesser Antilles Arc (https://freevectormaps.com/world-maps/caribbean/WRLD-CI-01-0001?ref=atr, last access: September 2021). (b) Topography of Barbuda Island in 2018 (after Irma), modified from Bain et al. (2018) and Brasier (1975a, b). (c) Codrington Lagoon morpho-bathymetry, from Brasier (1975a, b). (d) Codrington Lagoon morphology 3 months after Hurricane Irma from a satellite image of Barbuda obtained in December 2018 (© Google Earth Pro V 7.3.4.8248. December 2018. Barbuda; 17∘38′46.87′′ N, 61∘50′48.67′′ W; eye altitude 30 km. Landsat, Copernicus: 10 December 2021). The black dots correspond to sedimentary core locations sampled in March 2018 with their respective water depths. The two red lines correspond to seismic profiles recorded in June 2021.
2.1 Geomorphologic and geologic setting
Barbuda Island is located in the northeastern part of the Lesser Antilles Arc, 40 km north of Antigua Island (Fig. 1a). These two islands are surrounded by a large and up to 100 m deep carbonate platform called Barbuda Bank (Cornée et al., 2021). Barbuda Island is 13 km wide from east to the west and 22 km long from northwest to southeast (Fig. 1b). The highest point of the island reaches 39 m a.m.s.l. (above mean sea level) and is located in the so-called Highlands area (Weil-Accardo et al., 2022) (Fig. 1b). This low-lying island consists of coral limestone and is almost completely surrounded by fringing and barrier reefs, primarily along its eastern and northern coasts, whereas the western coast consists of patch reefs. The marine hydrodynamic regime in the island area displays a diurnal microtidal range, which is slightly more than 0.3 m throughout the year (Brasier, 1975a). The island displays a karstic limestone landscape containing numerous sinkholes and caves that retain freshwater throughout the year (Boger et al., 2020) and no surface streams (Potter et al., 2017). A series of saline ponds situated in the southern part of the island (Fig. 1b) are periodically flooded by spring tides and seawater during storms (Potter et al., 2017). The main permanent waterbody on the island is the hypersaline (measured at approximately 40 ‰ in 1970–1971; Stoddart et al., 1973) Codrington Lagoon (Fig. 1b–d). This lagoon lies in the western part of the island and is approximately 9 km long and 2.5 km wide. The Codrington Lagoon water depth ranges between 1 and 4.5 m, with a deeper part to the south (as observed in March 2018; Fig. 1c). This lagoon is separated from the ocean to the west by an approximately 1 to 3 m high (as observed in March 2018) and 140 to 260 m wide sandy barrier (Fig. 1c). This barrier is mostly made of coral debris, mollusks, calcareous algae and foraminifers and hosts a sparse hypersaline vegetation made of herbs and bushes. Prior to Hurricane Irma (September 2017), the barrier was continuous (Fig. 1c), and the northern part of the lagoon was connected to the ocean through a narrow channel, locally called “the creek” (Fig. 1b). After Hurricane Irma, two inlets developed (Fig. 1b and d). The eastern part of the lagoon is fringed by dense mangroves. The only human settlement on the island, the village of Codrington, is located on the eastern edge of the lagoon (Fig. 1b). The lagoon's bottom sediment is covered by seagrasses (i.e., Thalassia testudinum, whose root system can reach 80 cm below the sediment surface), algae and a few scattered coral species (Brasier, 1975a; Glumac and Curran, 2016; Potter et al., 2017).
2.2 Past hurricanes in Barbuda
Barbuda is located within the so-called Atlantic hurricane belt. Therefore, hurricanes are a major and frequent hazard, mostly between June and November (Boger et al., 2020). Many deposits related to past hurricanes were revealed by sedimentary studies along the coasts of various islands, in lagoons and sinkholes in this region (Donnelly and Woodruff, 2007; McCloskey and Keller, 2009; Malaizé et al., 2011; Denommee et al., 2015; Donnelly et al., 2015; Wallace et al., 2019; Biguenet et al., 2021; Winkler et al., 2022). Some paleotempestology studies have been conducted in Barbuda (Knowles, 2008; Burn et al., 2016), but none have been conducted in Codrington Lagoon. Burn et al. (2016) presented a reconstruction of Caribbean effective precipitation during the Little Ice Age, based on the study of the sediment infill of one of Barbuda's ponds (Freshwater Pond). Knowles (2008) analyzed transects of sediment cores from another of Barbuda's small ponds (Salt Pond) and identified overwash deposits and climate variability over the last 5000 years. Paleoclimate studies have shown that SSTs, storm intensity and storm frequency have varied through time in Barbuda (Brasier and Donahue, 1985; Khan et al., 2017; Knowles, 2008). Knowles (2008) identified three distinct periods: two active regimes of high-frequency storminess from 0–1500 and 3500–5000 yr BP, bracketing an inactive regime from 1500–3500 yr BP. The changes in the storminess frequencies are also influenced by many parameters, such as regional wind shear, which vary in response to the interannual migration of the Intertropical Convergence Zone (ITCZ), the Atlantic Multidecadal Oscillation (AMO) and the North Atlantic Oscillation (NAO) (Wallace et al., 2019). The oldest historically known hurricane that struck Barbuda Island occurred on 11 September 1813 (Oliver, 1894). The current observational record in the Lesser Antilles began in approximately 1850 CE. A total of 13 hurricanes passed within 30 km of Barbuda's Codrington Lagoon from 1859 to 2017 according to the National Oceanic and Atmospheric Administration (NOAA) (NOAA, 2021, Historical Hurricane Tracks) (Table 1, Fig. 2). Among the recent hurricanes that struck Barbuda Island, category 3 Hurricane Donna in 1960 (Dunn, 1961) and category 4 Hurricane Luis in 1995 (Hoggarth, 2001) were particularly destructive. Both caused significant damage to houses within the village of Codrington (Knowles, 2008). Hurricane Donna overwash deposit was probably recorded in Salt Pond (Knowles, 2008). Hurricane Luis breached a narrow strip of sedimentary barrier across Codrington Lagoon that was rapidly filled after the event (Potter et al., 2017). Low Bay Beach, located to the west of the Codrington Lagoon sandy barrier (Fig. 1b), experienced erosion after this event. The monitoring of this beach between 1996 and 2000 showed that erosion was dominant, with a mean profile area and erosion rate of −0.10 m2 and −0.80 m yr−1, respectively (James, 2003). Category 5 Hurricane Irma, which occurred in September 2017, was the latest and strongest hurricane that struck Barbuda (Table 1, Fig. 2). The hurricane reached Barbuda at its maximum intensity. The island was struck for more than 3 h by winds of 155 kn (287 km h−1) with maximum recorded gusts of wind speed of 360 km h−1, which directly caused the deaths of three people and the almost complete destruction of the structures on the island (Cangialosi et al., 2018). The minimum pressure record was 914 mb (Table 1). Hurricane Irma also induced a significant storm surge with a peak water level at 2.4 m mean higher high water (MHHW) recorded at a tide gauge located on Barbuda (Cangialosi et al., 2018). This storm surge induced a marine flooding with a runup reported to reach some 600 m inland (United Nations Development Programme, 2019).
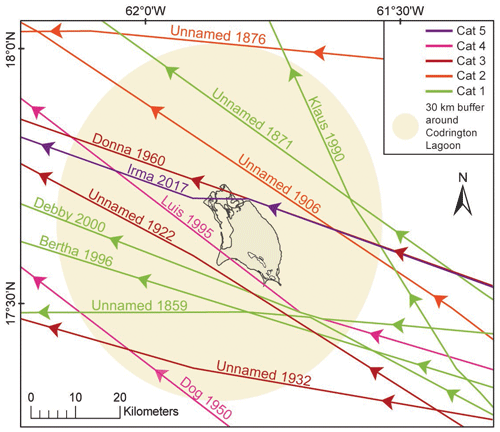
Figure 2Historical hurricane tracks (1859–2017) passing within 30 km of Codrington Lagoon (Barbuda) (NOAA, 2021, Historical Hurricane Tracks).
2.3 Other possible sources of overwashes in Barbuda: tsunamis
The Lesser Antilles Arc (Fig. 1a) marks the eastern boundary of the Caribbean Plate. This area is characterized by the subduction of the North American Plate under the Caribbean Plate (2 cm yr−1; DeMets et al., 2000). Thus, many active faults and volcanoes lie in the Lesser Antilles Arc and may generate tsunamis. During the historical period, only a few tsunamis were documented in the area (Zahibo and Pelinovsky, 2001; Lander et al., 2002; Harbitz et al., 2012; Engel et al., 2016). The primary sources for tsunamis were earthquakes (local, regional or far-field) (Reid and Taber, 1920; Pelinovsky et al., 2004; Zahibo et al., 2005; Roger et al., 2011; Harbitz et al., 2012; Cordrie et al., 2019; Biguenet et al., 2021) and volcanic processes (e.g., Pelinovsky et al., 2004; Watt et al., 2012). In the following, we describe major earthquake events and associated tsunamis that occurred locally in the Antigua–Barbuda area, then those that occurred regionally in the northern part of the Lesser Antilles, and then far-field events that hit the region. In the Antigua–Barbuda area, the event on 6 April 1690 CE is the oldest historically documented tsunami. It reached Antigua, Saint Kitts and Nevis, St. Thomas (US Virgin Islands), and Guadeloupe. This event was generated by an earthquake with an estimated magnitude of Ms 8 (Robson, 1964; Dorel, 1981; NGDC/WDS, 2020), and the epicenter was probably located between Nevis, Antigua and Montserrat (Feuillet et al., 2011). On 8 February 1843 CE, a small tsunami (wave amplitude of 1.2 m) was observed in English Harbor in Antigua and Nevis (Shepherd, 1992). The tsunami was generated by a M 7.5 to 8 earthquake (depending on the authors) between Antigua and Guadeloupe (Sainte-Claire Deville, 1843; Bernard and Lambert, 1988; Feuillet et al., 2011; Hough, 2013). More recently (8 October 1974), a large earthquake (Ms=7.1 to 7.6) occurred near Barbuda, north of Antigua, but did not generate any documented tsunami waves (Mccann et al., 1982). Apart from Barbuda itself, the oldest documented tsunami in the northern part of the Lesser Antilles region is a pre-Columbian tsunami (dated between 1200 and 1450 yr cal CE from 14C dating). It was identified within sedimentary cores sampled in Saint Thomas (US Virgin Islands; Fuentes et al., 2017), Anegada (British Virgin Islands; Atwater et al., 2017) and Scrub Island (Anguilla; Biguenet et al., 2021). The possible source for this tsunami is a magnitude 9 thrust earthquake located at the subduction interface facing Anegada Island (Cordrie et al., 2022). On 18 November 1867, another tsunami was documented in the Virgin Islands and Guadeloupe. This event was probably triggered by a strong earthquake in the British Virgin Islands (Reid and Taber, 1920), but its origin needs to be precisely defined (Zahibo et al., 2003). Finally, a transoceanic tsunami was observed in 1755 at 18 locations across the northeastern Caribbean from Bridgetown (Barbados) to Santiago de Cuba (Cuba) (NGDC/WDS, 2020). It was triggered by the well-known Mw 8 to 9 Lisbon earthquake (Chester, 2001).
3.1 Old nautical maps
The morphological evolution of Codrington Lagoon and its western sandy barrier was studied from a compilation of old nautical maps. The chosen maps were selected according to their date of publication. We chose the oldest map known (1750–1785 CE; Tweedy, 1981), the first accurate and detailed map known (1813 CE; Bain et al., 2018), and then two other maps illustrating the morphology of the island at the end of the 19th (Fullarton and Company, 1872) and 20th (Wigley, 1973) centuries. Although other maps of the island exist, they do not show significant morphological changes in Codrington Lagoon and its western sandy barrier compared to the selected maps.
3.2 Satellite images
The morphological evolution of the western sandy barrier of Codrington Lagoon over the period 1982–2021 was evidenced from a set of satellite images (Landsat and Global Land Survey data sets). All the images were downloaded from the Global Visualization Viewer of the US Geological Survey website (glovis.usgs.gov). The Global Land Survey (GLS) data sets were created by USGS and NASA using the primary Landsat sensor in use at the time for each collection epoch. The images were chosen according to their dates and the absence of clouds on the western sandy barrier. All the images have been resized to focus on Codrington Lagoon and facilitate the comparisons of the images. Our selection included (1) three Landsat 1–5 images from 1982 to 1992 using Multispectral Scanner (MSS) Level 1 images (processed to a 60 m pixel size); (2) one GLS2000 image from 1999, which uses Landsat 7 Enhanced Thematic Mapper Plus (ETM+), with a data resolution of 30 m; (3) two GLS2005 images from 2003 and 2006, which uses a combination of Landsat 7 ETM+ and Landsat 5 Thematic Mapper (TM), with a data resolution of 30 m; (4) one GLS2010 image from 2010, which uses a combination of Landsat 7 ETM+ and Landsat 5 TM, with a data resolution of 30 m; and (5) 11 Landsat 8 OLI (Operational Land Imager) images from 2013 to 2021, with 15 to 30 m resolution.
3.3 Very high-resolution seismic exploration
During June 2021, a seismic survey was carried out within Codrington Lagoon. A boomer plate associated with a line in a cone receiver, with a vertical resolution of 25 cm (IKB Seistec; Simpkin and Davis, 1993), was used to image the detailed internal architecture of the lagoon sediment fill. The shot interval was 250 ms, corresponding to an average distance between adjacent seismic traces of 0.125 m for a ship speed of 1 kn. Despite adverse weather conditions (strong winds between 18 and 40 kn and wind waves in the lagoon during the survey), 16 seismic profiles were recorded for a total length of 7131 m. Two seismic profiles displaying the typical internal architecture of the lagoon fill and new inlet are shown (profiles 13 and 15, respectively, Fig. 1d). The locations of the other seismic profiles can be found in the Supplement. Seismic data processing was carried out using Delph Seismic interpretation software, consisting of (1) noise mute in the water column, (2) swell filtering, (3) automatic gain correction and (4) bandpass filtering. We used a 1500 m s−1 P-wave velocity for the two-way travel time to depth conversion.
3.4 Sampling and core description
The sampling was carried out in March 2018 using an Uwitec gravity corer with a hammer. Six sediment cores of 60 to 130 cm were sampled in Codrington Lagoon at water depths of 3.2 to 4.5 m. The core locations were chosen along transects perpendicular to the lagoon's western sandy barrier. A south transect was conducted in front of the southern inlet (Fig. 1d), including from west to east cores BARB1-18-01 (no. IGSN TOAE0000000494), BARB1-18-02 (no. IGSN TOAE0000000495) and BARB1-18-03 (no. IGSN TOAE0000000496). A central transect was followed in front of the lagoon central inlet (Fig. 1d), including from west to the east cores BARB1-18-05 (no. IGSN TOAE0000000498) and BARB1-18-06 (no. IGSN TOAE0000000499). Finally, core BARB1-18-09 (no. IGSN TOAE0000000502) was taken just north of the central inlet (Fig. 1d). Cores have been split, described in detail, photographed and subsampled in the laboratory. The cores were horizontally stored in the laboratory walk-in cooler. Core locations and sampling procedures can be found in the French National Cyber Core Repository (https://cybercarotheque.fr/index.php, last access: September 2022).
3.5 Sedimentological analyses
For this study, sedimentological analyses were conducted on cores BARB1-18-01 and BARB1-18-05. Grain-size analyses were carried out at the EDYTEM laboratory using a Beckman Coulter LS 13320 XR particle size analyzer using sonication, with a range size between 0.010 and 3000 µm. The sampling step was approximately 1 cm through cores BARB1-18-01 (n=132) and BARB1-18-05 (n=123), respecting facies boundaries. No pretreatment was conducted on the samples. The shells larger than 2 mm were removed from every sample. Using the mean grain size to describe the samples was not appropriate here due to the high amount of shell debris, which tended to considerably increase the mean grain size. Thus, grain-size studies were restricted to particles smaller than 1 mm. The grain-size results were processed using an Efficient Mixed-Model Association (EMMA) algorithm implemented by QGrain software using the machine learning framework PyTorch (Liu et al., 2021). For core BARB1-18-01, an EMMA algorithm with three endmembers was chosen, as it best suited the core's main grain-size observations. The sum of the endmembers corresponded to 100 % of the grain-size frequency per sample. These three endmembers corresponded to the three main sediment grain-size modes: EM1 = 20 µm, EM2 = 127 µm and EM3 = 269–993 µm. For core BARB1-18-05, the EMMA algorithm that best suited the grain-size observation was the one with four endmembers. These four endmembers corresponded to the four main sediment grain-size modes: EM1 = 4 µm, EM2 = 20 and 50 µm, EM3 = 127 µm, and EM4 = 269–623 µm. Given that EM1 and EM2 of core BARB1-18-05 were similar to EM1 of core BARB1-18-01, we chose to add EM1 and EM2 of core BARB1-18-05. Thus, the grain-size results of cores BARB1-18-05 described and discussed below were EM1 = 4–18 µm and 50 µm, EM2 = 127 µm, and EM3 = 269–623. To simplify the grain-size descriptions for both cores in the paper, the different endmembers will be discussed as follows: EM1 is for clay to silt (4 to 50 µm), EM2 for fine sand (127 µm) and EM3 for medium to coarse sand (269 to 993 µm).
Core BARB1-18-01 was scanned using an X-ray tomography system (EasyTom XL 150 from RX SOLUTION society) at the SYMME laboratory (Annecy, France). The core was cut into two sections (126.5 to 70 cm and 70 to 0 cm). Each section was scanned at 150 kV and 66 µA for approximately 1 h 25 min to cover the entire volume of the core (8 successive scans from 126.5 to 70 cm and 10 successive scans from 70 to 0 cm). The images (16-bit.tif) obtained are cross sections of the cores (horizontal slices from top to base). For core BARB1-18-01 there were a total of 19 894 (8848+11 046) images available. Vertical cross sections of 63 µm resolution (voxel size) were reconstructed using ImageJ software with the Fiji distribution extension (Schindelin et al., 2012).
3.6 Geochemical analyses
The six cores were scanned at EDYTEM laboratory using the Avaatech Core Scanner (Avaatech XRF Technology, EDYTEM) with an Rh anode for X-ray fluorescence (XRF) analyses (Richter et al., 2006). For BARB1-18-01, two runs with a 1 mm step resolution were used to detect light- and heavy-weight elements: a run at 10 kV and 0.2 mA for 20 s and a run at 30 kV and 0.3 mA for 20 s with three replicates every 53 mm for both runs. For BARB1-18-02, two runs with a 1 mm step resolution were used: a run at 10 kV and 0.15 mA for 20 s and a run at 30 kV and 0.2 mA for 20 s with two replicates every 32 mm for both runs. For BARB1-18-03, two runs with a 2 mm step resolution were used: a run at 10 kV and 0.2 mA for 20 s and a run at 30 kV and 0.3 mA for 20 s with two replicates every 22 mm for both runs. For BARB1-18-05, two runs with a 2 mm step resolution were used: a run at 10 kV and 0.15 mA for 20 s and a run at 30 kV and 0.2 mA for 20 s with two replicates every 32 mm for both runs. For BARB1-18-06, two runs with a 2 mm step resolution were used: a run at 10 kV and 0.2 mA for 20 s and a run at 30 kV and 0.3 mA for 20 s with three replicates every 43 mm for both runs. For BARB1-18-09, two runs with a 1 mm step were used: a run at 10 kV and 0.1 mA for 20 s and a run at 30 kV and 0.15 mA for 20 s with two replicates every 22 mm for both runs. Element relative abundances are expressed as centered log ratios (CLRs) to avoid dilution effects due to water, for example (e.g., Weltje et al., 2015). These values were calculated using the equation from Weltje et al. (2015).
For core BARB1-18-01, the following elements (n=12) were used to calculate the geometric mean: Al, Si, S, Cl, K, Ca, Ti, Fe, Ni, Cu, Br and Sr. Na, Mg, P, Mn, Zn, Rb, Zr and Pb were not used because they display high uncertainties with a very noisy signal. For core BARB1-18-05, the following elements (n=15) were used to calculate the geometric mean: Na, Mg, Al, Si, Cl, K, Ca, Ti, Mn, Fe, Ni, Cu, Zn, Br and Sr. P, S, Rb, Zr and Pb were not used because of high uncertainties with a very noisy signal.
To highlight chemical endmembers (e.g., Sabatier et al., 2010b) and element correlations, principal component analysis (PCA) was performed on the raw XRF data of core BARB1-18-01 and BARB1-18-05 using the R software (R Core Team, 2021).
To quantify the amount of organic matter (LOI550) and carbonates (LOI950) in core BARB1-18-01 and BARB1-18-05, loss-on-ignition (LOI) analyses were performed following the protocol described by Heiri et al. (2001). The sampling step was 4 cm (n=33 and n=31). Biguenet et al. (2021) showed that for carbonate-rich sediments, the 2 h at 950 ∘C proposed in the protocol was not sufficient to burn all carbonates. Here, the carbonate content in the samples was 3 % to 17 % higher after a second burn at 950 ∘C.
Slabs (8.5×1.5 cm) were sampled from cores BARB1-18-01 (n=6) and BARB1-18-05 (n=4) to be resin-embedded. The small-scale sedimentary structures and grains of the slabs were observed with a Leica LEO Stereoscan 440 (ASTRE platform of the Université Savoie Mont-Blanc) scanning electron microscope (SEM) with 20 kV tension and at a 10 mm work distance. The elementary composition at the grain scale was highlighted through chemical maps that were performed on the slabs at a 24 mm work distance using the QUANTAX EDX probe (Bruker) from the ASTRE platform. The images were obtained in high-vacuum mode in secondary electron or backscattering electron modes.
3.7 Foraminifera analyses
To analyze the sediment foraminifera content, samples were taken every 5 to 10 cm and every 10 cm according to sedimentary facies boundaries in cores BARB1-18-01 (n=20) and BARB1-18-05 (n=13). Samples were weighed and dried at 60 ∘C. The dried residues were sieved to retain the foraminifera from the 125–500 and >500 µm fractions. Individuals were identified at least at the genus level using common taxonomical reference literature describing the foraminifera in the Gulf of Mexico and the Caribbean Sea. To determine the foraminifera abundance per sample, a semi-quantitative scale was elaborated based on the number of specimens observed in a single 6×10 cm picking tray filled with dry residue.
3.8 Chronology
Short-lived radionuclides (210Pb, 137Cs) were measured using well-type Ge detectors at the Laboratoire Souterrain de Modane (methods from Reyss et al., 1995). Samples were collected from cores BARB1-18-01 (n=23) and BARB1-18-05 (n=21) in the upper 34 and 30 cm, respectively, according to lithological variations. The difference between total 210Pb and 226Ra activities was attributed to excess 210Pb (“210Pbex”) (Goldberg, 1963). Results were calculated using the package “serac” in the R software (Bruel and Sabatier, 2020). Organic macroremains (wood, leaves and roots) were collected in the six cores (n=21). The 14C measurements were carried out by accelerator mass spectrometry (AMS) at the Laboratoire de Mesure du Carbone 14 (LMC14), ARTEMIS, at the CEA (Atomic Energy Commission) institute at Saclay and at the Poznan Radiocarbon Laboratory. The 14C ages were calibrated at 2σ using the IntCal20 calibration curve (Reimer et al., 2020).
4.1 Codrington Lagoon sandy barrier morphological evolution from the 18th century to 2021
The compilation of old Barbuda nautical maps (Fig. 3) argues in favor of relatively few morphological changes throughout the period when compared to today's island coastal geomorphology (Fig. 1b). The oldest map known of the island (1750–1785 CE, Fig. 3a), although poorly representing the proportions of the island, displays valuable information about the Codrington Lagoon sandy barrier. The “large pond”, which corresponds to the current Codrington Lagoon, is completely isolated from the open ocean, apart from the narrow channel located in its north (the creek). The three other maps (1813 CE, Fig. 3b; 1872 CE, Fig. 3c; 1973 CE, Fig. 3d) also display a continuous western sandy barrier.
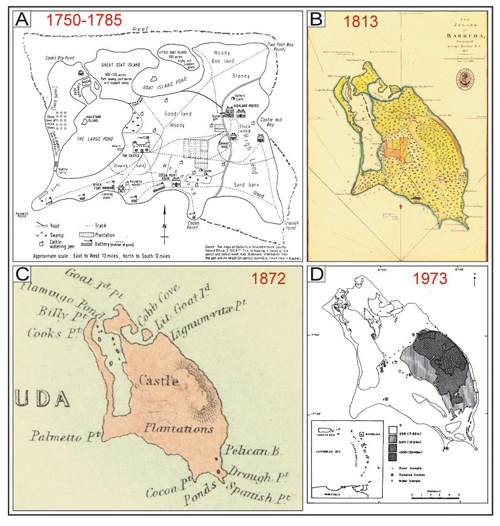
Figure 3Barbuda old nautical maps. (a) Two maps of Barbuda in Gloucestershire County. Record Office D1610 P17. This redrawing is based on the pencil and color-wash map. Additional information from the pen and ink version (of identical outline) is shown here in brackets, from Tweedy (1981). (b) The island of Barbuda, surveyed in 1813 by Capt. R. N. Deckar, Hydrographical Office (PRO, CO 700, MP/8 Antigua X/04800), from Bain et al. (2018). (c) The West Indies, by G. H. Swanston, Edinr. (with) The Most Important Of The Lesser British Islands On An Enlarged Scale. LXI. Engd. by G. H. Swanston Edinburgh. A. Fullarton & Co. Edinburgh, London and Dublin, from David Rumsey Map Collection (https://www.davidrumsey.com/, last access: October 2021). (d) Barbuda, location and elevation map, from Wigley (1973).
The detailed morphological evolution of the western barrier can be outlined from 1982 onwards using satellite images (Fig. 4). The barrier was thin but continuous in 1982 and 1986. The barrier remained continuous in 1990 and 1992, although Hurricane Klaus (H1) struck Barbuda in 1990. The first exploitable satellite image after hurricanes Luis (H4, 1995) and Bertha (H1, 1996) was obtained in 1999. This image shows that the extreme south of the barrier widened toward the lagoon side (circled in red on the image), displaying a washover morphology. Nevertheless, no breach is observable. The barrier was still continuous in the 2003 satellite image, although Hurricane Debby (H1) struck Barbuda in 2000. The washover observed in 1999 was still visible in February 2017 and was smaller in August 2017. The first usable satellite image after Hurricane Irma, which struck Barbuda Island in September 2017, is from November 2017. Two inlets are observed: a 1.2 km wide inlet in the center of the barrier and a 0.5 km wide inlet in the southern part of the barrier. From March to July 2018 (6 to 10 months after the event), the southernmost inlet was filled by sediment. However, the central inlet has been enlarged since its formation in 2017 (1.9 km wide in September 2021).
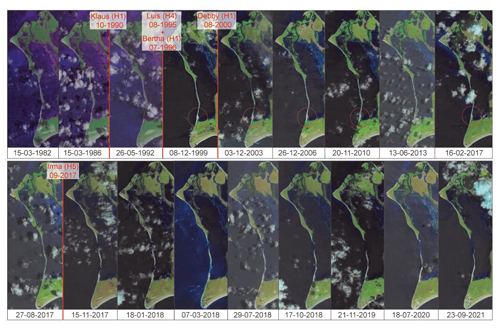
Figure 4Satellite images displaying morphological evolutions of the Codrington Lagoon western sandy barrier over the period 1982–2021 (Landsat and Global Land Survey data sets). The images in chronological order are as follows (from glovis.usgs.gov): Landsat 1–5 MSS C1 Level-1 LM03_L1GS_002048_19820315_20180413_01_T2; Landsat 1–5 MSS C1 Level-1 LM05_L1GS_002048_19860315_20180331_01_T2; Landsat 1–5 MSS C1 Level-1 LM04_L1TP_002048_19920526_20180318_01_T2; Global Land Survey P002R048_7X19991208; Global Land Survey LE70020482003337EDC03; Global Land Survey LE70020482006057EDC00; Global Land Survey LE70020482010324ASN00; Landsat 8 OLI/TIRS C1 Level-1 LC08_L1TP_002048_20130613_20170504_01_T1; Landsat 8 OLI/TIRS C1 Level-1 LC08_L1TP_002048_20170216_20170228_01_T1; Landsat 8 OLI/TIRS C1 Level-1 LC08_L1TP_002048_20170827_20170914_01_T1; Landsat 8 OLI/TIRS C1 Level-1 LC08_L1TP_002048_20171115_20171122_01_T1; Landsat 8 OLI/TIRS C1 Level-1 LC08_L1TP_002048_20180118_20180206_01_T1; Landsat 8 OLI/TIRS C1 Level-1 LC08_L1TP_002048_20180307_20180320_01_T1; Landsat 8 OLI/TIRS C1 Level-1 LC08_L1TP_002048_20180729_20180814_01_T1; Landsat 8 OLI/TIRS C1 Level-1 LC08_L1TP_002048_20181017_20181030_01_T1; Landsat 8 OLI/TIRS C1 Level-1 LC08_L1TP_002048_20191121_20191203_01_T1; Landsat 8 OLI/TIRS C1 Level-1 LC08_L1TP_002048_20200718_20200722_01_T1; Landsat 8 OLI/TIRS C1 Level-1 LC08_L1TP_002048_20210923_20210923_01_RT. The red bars correspond to hurricanes that passed within 30 km of Codrington Lagoon during the visualized period. H1, H2 and H5 indicate the hurricane category on the Saffir–Simpson hurricane wind scale.
A very high-resolution seismic profile, recorded in June 2021 within the large central inlet opened after Hurricane Irma, allows us to find evidence of morphological changes in cross sections (Profile 15, Fig. 5). This profile displays two main unconformities (Fig. 5). The basal unconformity EU0 (in green, Fig. 5b) corresponds to an irregular, subhorizontal erosional surface, clearly evidenced by the truncation of underlying reflectors. The upper unconformity EUi (in pink, Fig. 5b) is subhorizontal and less irregular than EU0. EUi is evidenced by downlaps of the overlying reflectors, whereas erosional truncations of underlying reflectors are weakly expressed. The basal unit U0, which lies under EU0, displays medium-amplitude, low-continuity and medium-frequency reflectors (Fig. 5b). Some reflectors within U0 are subhorizontal to gently inclined. Most of this unit is chaotic to transparent. The low penetration of acoustic waves prevents detailed observations within U0. The outer shape of seismic Ui1, i.e., lying between EU0 and EUi, is a sheet drape with thicknesses ranging from 0.7 to 1.8 m (Fig. 5b). It displays low-amplitude, medium-continuity and medium-frequency reflectors. The reflectors in Ui1 are mostly subhorizontal and gently inclined toward the lagoon in the middle part of the profile. The outer shape of the uppermost unit Ui2 consists of a 1 m thick sheet drape to the east and to a bank to the west (Fig. 5b). The maximum bank thickness reaches more than 2.5 m. It displays reflectors with medium to strong amplitude, good continuity, and medium to low frequencies. Reflectors within the eastern part (i.e., lagoonward) of Ui2 are subhorizontal, whereas they are mounded within the western part (i.e., oceanward) of Ui2.
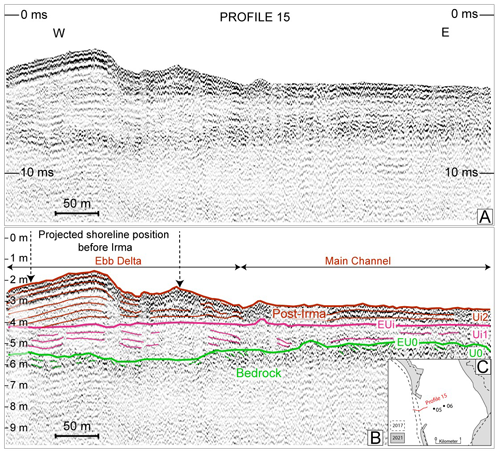
Figure 5(a) Seismic profile 15 (length approximately 570 m) showing a part of the lagoon central inlet structure (east–west transect) in June 2021. (b) Interpreted profile. (c) Profile localization in comparison with the sandy barrier in 2021 (in gray) and in 2017 before Hurricane Irma (in dotted lines).
4.2 Sedimentary record in Codrington Lagoon
4.2.1 Seismic stratigraphy of Codrington Lagoon
The internal architecture of Codrington Lagoon is illustrated by seismic Profile 13 (Fig. 6), in which three main unconformities are observable. The lowest one, EU0 (in green, Fig. 6b), corresponds to an irregular, subhorizontal erosional surface, clearly evidenced by the truncation of underlying reflectors. The middle unconformity EUl1 is subhorizontal to gently inclined toward the west (in blue, Fig. 6b). This is evidenced by downlapping of the overlying reflectors. The upper unconformity EUl2 is subhorizontal (in orange, Fig. 6b). This is evidenced by toplaps of the underlying reflectors. The basal unit U0 lying under EU0 displays medium-amplitude, medium-continuity and medium-frequency reflectors (Fig. 6b). Reflectors within U0 are subhorizontal. The outer shape of seismic Ul1 lying between EU0 and EUl1 is a sheet drape with thicknesses ranging from 0.6 to 2 m (Fig. 6b). One strong-amplitude reflector, gently inclined to the west, is observed within Ul1. Seismic unit Ul2, which lies between EUl1 and EUl2, displays reflectors with low to medium amplitude and medium continuity (Fig. 6b). These reflectors dip to the west and display a shingled internal configuration. Seismic unit Ul3, lying on EUl2, displays medium-amplitude and subhorizontal reflectors with good continuity in the western part of the profile (Fig. 6b). To the east, Ul3 displays low-amplitude chaotic reflectors. The sea bottom is marked by three parallel reflectors that likely correspond to an artifact, as they are frequently observed in very shallow exploration on sandy bottoms (Chaumillon et al., 2008). Seismic profile 13 was set to cross the position of three sediment cores taken in 2018: BARB1-18-01, 02 and 03 (Fig. 6). This allowed us to sample sediments within seismic unit Ul3. BARB1-18-02 reached the top seismic unit, Ul2, below Ul3.
4.2.2 Sediment core lithological description
Three main sedimentary facies are distinguishable from the six cores sampled in Codrington Lagoon. These facies are all included in seismic unit Ul3 (Fig. 6b). Only core BARB1-18-02 may have reached underlying unit Ul2. In the following, sedimentary facies are mainly described from cores BARB1-18-01 and BARB1-18-05.
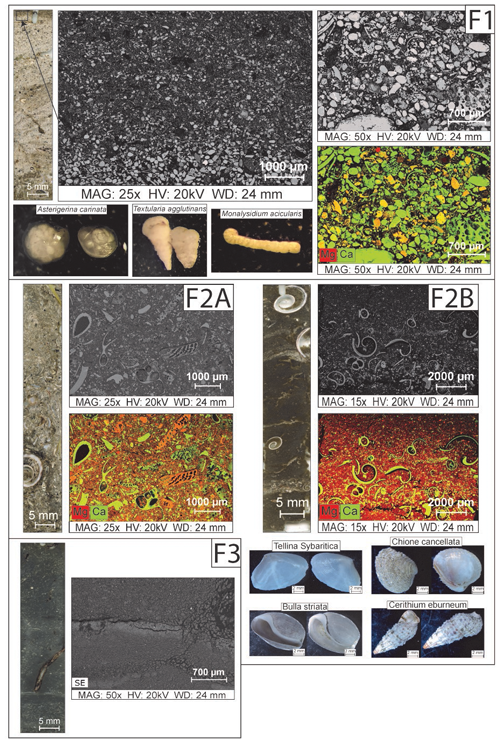
Figure 7Main facies of the six cores from Codrington Lagoon. For every facies (F1, F2A, F2B and F3), there is a picture of a thin slide on the left, SEM images and geochemical cartographies (with Ca in green, Mg in red) from cores BARB1-18-01 and BARB1-18-05. In F1 and F2, there are optical microscope photographs of foraminifera (<125 µm) and bivalve and gastropod shells, respectively, taken from cores BARB1-18-01 and BARB1-18-05.
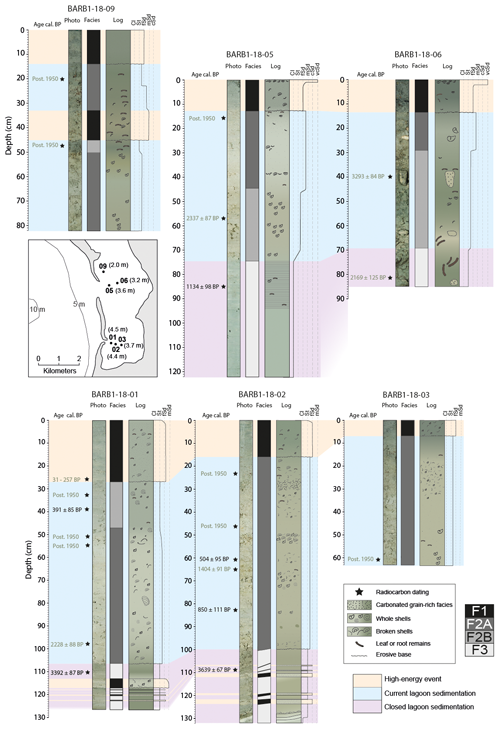
Figure 8Photos, facies logs and sedimentary logs for cores BARB-18-01, 02, 03, 05, 06 and 09 with the depth of the 14C ages (in cal BP) (indicated with black stars). The most reliable 14C ages are written in black, and the others are shown in gray. The orange, blue and violet lines highlight the different types of sedimentary deposits that were correlated between cores. The indicated depths are relative to the bottom of the lagoon. The cores' location and water depths are indicated on the map.
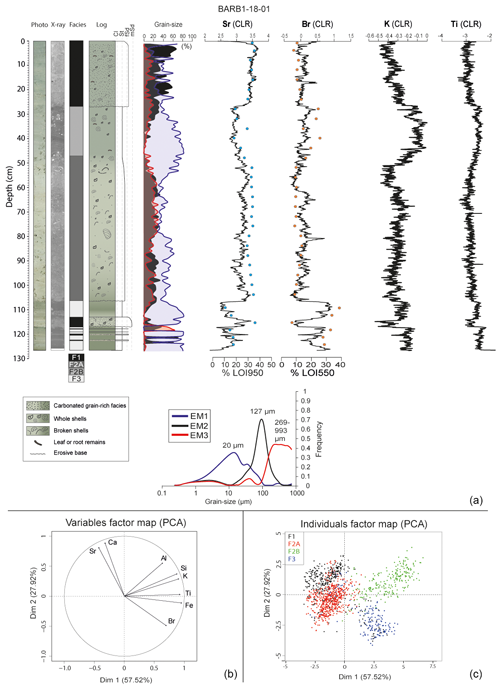
Figure 9Geochemical and grain-size data for core BARB1-18-01. (a) From left to right, picture, X-ray, facies log, lithological description, grain-size endmember distributions and their corresponding grain-size modes underneath, and centered log ratio (CLR) XRF data for Sr, Br, K and Ti. The LOI950 and LOI550 percentages are superimposed on the Sr and Br data, respectively. (b) PCA of the XRF data with the grain-size endmembers. (c) Map of the geochemical data distribution with facies information. The black dots correspond to F1, the red dots to F2A, the green dots to F2B and the blue dots to F3.
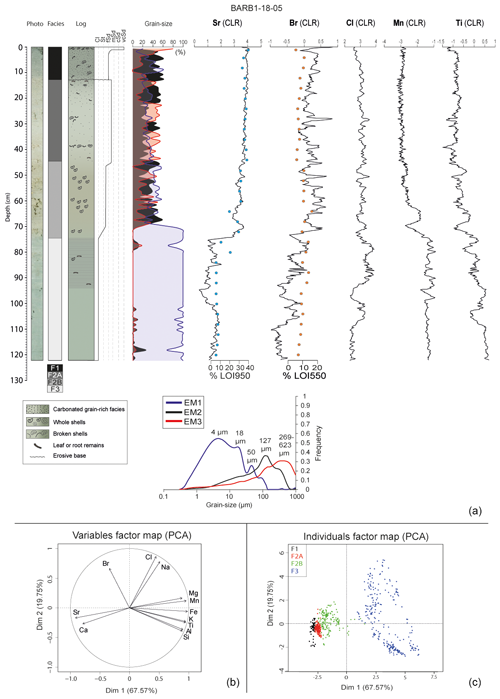
Figure 10Geochemical and grain-size data for core BARB1-18-05. (a) From left to right, picture, X-ray, facies log, lithological description, grain-size endmember distributions and their corresponding grain-size modes underneath, and centered log ratio (CLR) XRF data for Sr, Br, Cl, Mn and Ti. The LOI950 and LOI550 percentages are superimposed on the Sr and Br data, respectively. (b) PCA of the XRF data with the grain-size endmembers. (c) Map of the geochemical data distribution with facies information. The black dots correspond to F1, the red dots to F2A, the green dots to F2B and the blue dots to F3.
Facies 1 (F1) consists of light gray to light olive gray fine sand deposited at the top of the six cores (Figs. 7 and 8). F1 thickness varies from 7 to 27 cm in cores BARB1-18-03 and BARB1-18-01, respectively, and becomes thinner with increasing distance from the sandy barrier (Fig. 8). A medium-sand F1 layer is also visible in core BARB1-18-09 from 45 to 33 cm depth (Fig. 8). F1 is also observed within five thin layers at the bottom of cores BARB1-18-01 and BARB1-18-02 (0.25 to 4 cm thick) intercalated with Facies 3 (see below). The core BARB1-18-01 X-ray tomography scan highlights these denser layers of fine sand (lighter gray, Fig. 9a). The two deepest layers in core BARB1-18-01 were correlated to two layers in core BARB1-18-02 (Fig. 8). F1 layers are generally characterized by sharp basal contact with the underlying facies. Grain-size endmember analyses of F1 within core BARB1-18-01 show a mean repartition of 24 % silt (20 µm), 49 % fine sand (127 µm) and 27 % medium to coarse sand (269–993 µm) (Fig. 9a). Grain-size endmembers of F1 within core BARB1-18-05 show a mean repartition of 31 % clay to silt (4–18 and 50 µm), 46 % fine sand (127 µm) and 23 % medium to coarse sand (269–623 µm) (Fig. 10a). Thus, fine sand (127 µm) is the main grain-size mode observed in F1. F1 consists of 36.5 (±2.5) % carbonates (LOI950) and 8.5 (±2) % organic matter (LOI550) in cores BARB1-18-01 and BARB1-18-05 (Figs. 9a and 10a). F1 is made of carbonate sand. The sand originates from various eroded fragments of marine skeletal material (e.g., corals, mollusks, bivalves and foraminifers). Many shells fragment of variable sizes (100 to 1000 µm) are usually mixed with the sand and sometimes form a broken shell layer at the top of the F1 deposit (e.g., cores BARB1-18-05 and BARB1-18-06, Fig. 8). The F1 deposits' carbonate grains (sand grains and shell fragments) are composed of calcite and magnesium calcite (Fig. 7). F1 displays the highest foraminifera diversity in cores BARB1-18-01 and BARB1-18-05, with 14 to 25 different species identified per sample. This rich and intact fauna is dominated by an abundance of individuals (6 to more than 41 individuals per species in samples from the semiquantitative abundance study) of the symbiont-bearing taxon Archaias angulatus, the Miliola sandy taxon Pseudotriloculina rotunda and many Quinqueloculina species. The robust-shaped A. angulatus is known to live in coarse sand, both inside Codrington Lagoon and from the shore zone to the forereef around the island (Brasier, 1975b). P. rotunda is a sessile and encrusting taxon that thrives near dense vegetation, such as Thalassia testudinum, both inside the lagoon and in reefs around Barbuda (Brasier, 1975a, b). Among the many species found in F1, some were restricted to this facies. These rare (one to two individuals per species in samples), very slightly damaged individuals, were found in samples from the F1 layer deposited at the top of BARB1-18-01 and BARB1-18-05. These individuals form part of unidentified species, such as Pyrgo sp. 1, Elphidium sp. 1, Bolivina sp. 1 and Amphistegina sp., but also Asterigerina carinata, Pseudohauerinella occidentalis, Textularia agglutinans, Cymbaloporetta squammosa and Monalysidium acicularis. A. carinata (Fig. 7) is known to live outside of Codrington Lagoon in vegetated bottoms of the marine area west of the present-day sandy barrier (Brasier, 1975b). P. occidentalis is found in tropical back-reef and reef environments (Javaux and Scott, 2003). T. agglutinans (Fig. 7) is known to live in the forereef around Barbuda Island (Brasier, 1975b). C. squammosa is a sessile and encrusting taxon that lives in vegetated areas of the reef and interreef around Barbuda Island (Brasier, 1975b). M. acicularis (Fig. 7) is known to live abundantly in the high intertidal area of Shuaiba Lagoon (eastern Red Sea; Abu-Zied and Bantan, 2013).
Facies 2 (F2) is divided into two alternating subfacies: Facies 2A (F2A) and Facies 2B (F2B) (Figs. 7 and 8). The depths and thicknesses of these subfacies vary between cores, revealing lateral facies variations in the lagoon (Fig. 8). F2A consists of light gray to grayish brown sandy silt, with thicknesses varying from 16 to 86 cm in BARB1-18-06 and BARB1-18-02, respectively (Figs. 7 and 8). Grain-size endmembers of F2A within core BARB1-18-01 show a mean repartition of 55 % silt (20 µm), 25 % fine sand (127 µm) and 20 % medium to coarse sand (269–993 µm) (Fig. 9a). Grain-size endmembers of F2A within core BARB1-18-05 show a mean repartition of 30 % clay to silt (4–18 and 50 µm), 34 % fine sand (127 µm) and 36 % medium to coarse sand (269–623 µm) (Fig. 10a). Thus, silt (20 µm) is the grain-size mode that describes F2A the best for core BARB1-18-01. The three grain-size endmembers of core BARB1-18-05 display almost equivalent percentages, showing a mix of the three main grain-size populations, with the highest percentage being the medium to coarse sand (269–623 µm). F2A consists of 36.5 (±1.5) % carbonates (LOI950) and 8 (±1.5) % organic matter (LOI550) in cores BARB1-18-01 and BARB1-18-05 (Figs. 9a and 10a). F2A consists of a mix of silt and carbonate sand. The sand originates from various eroded fragments of marine skeletal material (e.g., corals, mollusks, bivalves and foraminifers). Many shell fragments of variable sizes (100 µm to several centimeters) are usually mixed with the sand. The carbonate grains contain Mg-rich calcite (Fig. 7).
F2B consists of gray sandy silt, with thicknesses varying from 5 to 30 cm in BARB1-18-09 and BARB1-18-06, respectively (Figs. 7 and 8). This facies is absent from cores BARB1-18-02 and BARB1-18-03. Grain-size endmembers of F2B within core BARB1-18-01 show a mean repartition of 81 % silt (20 µm), 11 % fine sand (127 µm) and 8 % medium to coarse sand (269–993 µm) (Fig. 9a). Grain-size endmembers of F2B within core BARB1-18-05 show a mean repartition of 52 % clay to silt (4–18 and 50 µm), 30 % fine sand (127 µm) and 18 % medium to coarse sand (269–623 µm) (Fig. 10a). Thus, silt is the grain-size mode that describes F2B the best for both cores, with a higher percentage in core BARB1-18-01, as seen in F2A. F2B consists of 28 (±4) % carbonates (LOI950) and 15 (±3) % organic matter (LOI550) in cores BARB1-18-01 and BARB1-18-05 (Figs. 9a and 10a). It is mainly composed of a silty Mg-rich calcite matrix with almost undamaged shells of variable sizes (100 µm to several centimeters) (Fig. 7). The carbonate grains (sand grains and shells) are made of calcite (Fig. 7). F2A and F2B both display 5 to 18 different foraminifera species per sample in cores BARB1-18-01 and BARB1-18-05. This fauna is also dominated by an abundance of individuals (6 to more than 41 individuals per species in samples) of A. angulatus, P. rotunda and many Quinqueloculina species. Furthermore, F2A and F2B display many whole and intact bivalve and gastropod shells, with the shells from F2A showing more wear. These shells are of variable sizes (1 to 5 cm) (Fig. 7). The identified individuals found in the sediment were Bulla striata, Cerithium eburneum, Chione cancellata and Tellina sybaritica, which are known to live in Codrington Lagoon (Brasier and Donahue, 1985).
Facies 3 (F3) consists of gray to light olive gray clay to silt, located at the core bases with thicknesses varying from 16 to 47 cm in cores BARB1-18-06 and BARB1-18-05, respectively (Figs. 7 and 8). The boundary between Facies 3 and Facies 2 in cores BARB1-18-01 and BARB1-18-02 is correlated with the strong amplitude reflector in Ul3 (in red, seismic profile 13, Fig. 6b). Facies 3 is absent from the shorter cores BARB1-18-03 and BARB1-18-09. Grain-size endmembers of F3 within core BARB1-18-01 show a mean repartition of 92 % silt (20 µm), 7 % fine sand (127 µm) and 1 % medium to coarse sand (269–993 µm) (Fig. 9a). Grain-size endmembers of F3 within core BARB1-18-05 show a mean repartition of 97 % clay to silt (4–18 and 50 µm), 2 % fine sand (127 µm) and 1 % medium to coarse sand (269–623 µm) (Fig. 10a). Thus, F3 is almost exclusively composed of fine sediment (clay to silt) for both cores. The slightly higher percentage of fine sand (127 µm) in core BARB1-18-01 can be explained by the presence of thin F1 layers at its base. F3 is very homogenous with almost nothing apart from the clay to silt matrix. It is composed of 14 (±6) % carbonates (LOI950) and 19 (±8) % organic matter (LOI550) in cores BARB1-18-01 and BARB1-18-05 (Figs. 9a and 10a). A total of 0 to 11 different foraminifera species were identified per sample in F3 from cores BARB1-18-01 and BARB1-18-05. Facies 3 is dominated by many Quinqueloculina species (1 to 15 individuals per species in samples).
4.2.3 Geochemistry
The main BARB1-18-01 XRF core scanning results are shown in Fig. 9. A moderate relationship is shown between Sr linear regression tests and LOI950 results, with an R value of 0.45 and p value < 0.00003853. These two indicators present the same long-term variation, but this weak relation probably indicates a variable source of Sr, usually found in carbonate shells of micro- and macrofauna. A significant relationship is shown between Br linear regression tests and LOI550 results, with an R value of 0.86 and p value < . As Br is known to have a strong affinity for organic matter (e.g., Chagué-Goff and Fyfe, 1996; Bajard et al., 2016; Biguenet et al., 2021), this suggests that Br (CLR) can be used as a high-resolution proxy for organic matter content. F3 simultaneously displays relatively low Sr (CLR) values and high Br (CLR) values, indicating a decrease in carbonate and an increase in organic matter contents (Fig. 10a). F3 also displayed the highest value for Ti (CLR) (Fig. 9a).
The total variance is represented at 85 % by the first two dimensions (Dim1 and Dim2) of the PCA (Fig. 9b). Al, Si, K, Ti, Fe and Br show a positive loading on Dim1. Sr and Ca demonstrate a low negative loading on Dim1. Fe and Br (negative loading) can be distinguished from Al, Si, K and Ti (positive loading) on Dim2. Sr and Ca also show a high positive loading on Dim2, displaying a negative correlation with Br. This PCA representation allows us to identify three different geochemical endmembers: (1) “carbonate” elements illustrated by Ca and Sr; (2) “organic-matter”-related elements (OM) represented by Br; and (3) “silicate” allochthonous inputs represented by Al, Si, K, Fe and Ti. A map of the geochemical data distribution was established from labeling samples with the facies information in the PCA (Fig. 9c). F1 is controlled by the “carbonates” endmember because of the numerous shell fragments with high concentration in Sr and Ca in this facies (e.g., Sabatier et al., 2010b; Chagué-Goff et al., 2017; Biguenet et al., 2021). F2A is also influenced by the “carbonates” endmember with a negative loading on Dim1. F2B seems to be correlated with the “silicates” endmember, while F3 is more influenced by the “organic matter” and to a lesser extent by the “silicates” endmember.
The main BARB1-18-05 XRF core scanning results are shown in Fig. 10. A highly significant relationship is shown between Sr linear regression tests and LOI950 results, with an R value of 0.86 and p value < . Given that Sr is mostly found in carbonate shells of micro- and macrofauna, its variations could be used as a high-resolution proxy for carbonate fauna content in the sediment. A weaker relationship compared to core BARB1-18-01 is shown between Br linear regression tests and LOI550 results, with an R value of 0.43 and p value < 0.00004188. The lower LOI550 values (<20 %, Fig. 10a) of core BARB1-18-05 may explain the weaker relationship between Br and LOI550 compared to core BARB1-18-01. F3 displayed the highest value for Ti (CLR) (Fig. 10a).
The total variance is represented at 87 % by the first two dimensions (Dim1 and Dim2) of the PCA (Fig. 10b). Cl, Na, Mg, Mn, Fe, K, Ti, Al and Si show a positive loading on Dim1. Ca, Sr and Br have a negative loading on Dim1. Br, Na, Cl, Mg and Mn demonstrate a positive loading on Dim2. Ca, Sr, Fe, K, Ti, Al and Si display a negative loading on Dim2. This PCA representation allows us to identify four different geochemical endmembers: (1) “carbonates” illustrated by Ca and Sr; (2) “organic matter” (OM) represented by Br; (3) “evaporites” represented by Na, Cl and Mg; and (4) “silicates” represented by Fe, K, Ti, Al and Si. A map of the geochemical data distribution was established from labeling samples with the facies information in the PCA (Fig. 10c). F1 and F2A are strongly linked to the “carbonates” endmember. F2B is dominated by the “organic matter” endmember and not by the “silicates” endmember, as for core BARB1-18-01. F3 is influenced by both the “evaporites” and “silicates” endmembers, as for core BARB1-18-01.
Thus, it appears that F1 and F2A are strongly linked with the “carbonates” endmember in both cores. F2B is more associated with the “silicates” endmember in core BARB1-18-01 and with the “carbonates” endmember in core BARB1-18-05. F3 is more associated with the “organic matter” endmember in core BARB1-18-01 and with the “evaporites” and “silicates” endmembers in core BARB1-18-05.
4.2.4 Chronology
Short-lived radionuclides
The sedimentation rate could not be calculated from 210Pbex activities for these cores. With the exception of the topmost sample in core BARB1-18-01, the F1 layer in both core tops displays little variation in 210Pbex with relatively low values (between 34 and 63 mBq g−1 for core BARB1-18-01 and between 53 and 72 mBq g−1 for core BARB1-18-05), indicating that this layer was probably related to an event deposit (Bruel and Sabatier, 2020) (Fig. 11). Moreover, an intermediate value of 210Pbex (13 mBq g−1 at 26 cm depth in core BARB1-18-01 and 27 mBq g−1 at 12 cm depth in core BARB1-18-05), lower than those in F1 and higher than those in the underlying sediment, was measured in both profiles at the base of F1. These intermediate activities may indicate the mixing of sediment from different ages and thus erosion at the base of the F1 deposit in both cores. The topmost 210Pbex activity sample (0 to 0.5 cm depth) in BARB1-18-01 displays a higher value, which could indicate present-day sedimentation after the deposition of the homogeneous, thick F1 layer. There is no clear 137Cs activity in cores BARB1-18-01 and BARB1-18-05 (Fig. 11). The highest 137Cs activity on one sample in the homogeneous F1 event indicates that this layer is probably related to post-nuclear-fallout sediment remobilized in this event deposit. This allows us to date the F1 deposit to after 1963 CE.
14C ages
The oldest 14C sample in sediments cored within the lagoon yields a calibrated age between 3570 and 3704 cal BP at the base of core BARB1-18-02 (108.5 cm, Table 2). Generally, the 14C dating results show many dates after 1950 and many inversions of ages. Two hypotheses can be proposed to explain these results: (1) the possibility that some of the plant macroremains used for 14C dating were debris of Thalassia testudinum roots, whose root system can reach 80 cm below the sediment surface resulting in 14C ages much younger than the sediment at the same depth, and (2) the strong hydrodynamics in the lagoon could enable the erosion and transport of old debris and mix them with younger sediment. These complex 14C results prevented the construction of an age model for the cores. Nevertheless, a correlation based on sediment facies boundaries was tentatively made between the six sampled cores (Fig. 8). The bases of cores BARB1-18-01 and 02 were easily correlated with two similar thin F1 layers intercalated in F3 and a sharp boundary between F3 and F2 (Fig. 8). The 14C ages located at the base of these two cores are also coherent (>3000 cal BP). Thus, we decided to trust the ages located in F3 for cores BARB1-18-01 and 02. The ages near the F3-F2 boundary in cores BARB1-18-05 and 06 are different: 1059–1255 and 2057–2306 cal BP, respectively. As core BARB1-18-05 displays a very homogenous clay without any debris in F3 and BARB1-18-06 shows more debris and plant macroremains (possibly Thalassia testudinum roots), we choose to trust the age in core BARB1-18-05 (1059–1255 cal BP). Consequently, it appears that the F3–F2 boundary was probably not synchronous in all the lagoon, with a later boundary in the central part compared to the southern part of the lagoon.
5.1 Facies interpretations and paleoenvironmental reconstitutions
Even though it was not possible to establish an accurate age model, available ages and contrasted facies successions allow us to reconstitute environmental changes that occurred in Codrington Lagoon over the last 3 millennia.
Facies 3 (F3) is located at the core bases and displays the finest grain size (clay to silt), recording a lower-energy and more sheltered environment than the present-day lagoon. As Barbuda Island is purely carbonate, the highest values of silicates in F3 are most likely related to long-range-transported aeolian particles brought via summer easterly trade winds from Africa to this island (Kumar et al., 2018), similar to what was observed over the Virgin Islands (Kumar et al., 2018) and in a lagoon of Scrub Island, located 120 km to the northwest (Biguenet et al., 2021). The highest values of silicates in F3 can be explained by smaller silicate dilution compared to other facies because of a lower sedimentation rate. F3 shows the lowest carbonate percentage (LOI950) and low Sr (CLR) content in cores BARB1-18-01 and 05 and a very low diversity and quantity of foraminifera, indicating low carbonate production. F3 in core BARB1-18-01 displays a high content of organic matter (represented by Br). In contrast, F3 in core BARB1-18-05 displays high contents of evaporitic salts (represented by Na, Cl and Mg) and silicates (represented by Fe, K, Ti, Al and Si). Brasier (1975a) showed that hypersaline lagoons generally display low-foraminifera standing crops. Thus, the high Cl (CLR), low Sr (CLR) and low quantity and diversity of foraminifers in core BARB1-18-05 can be interpreted as records of a hypersaline environment, suggesting that connections between Codrington Lagoon and the ocean were reduced during F3 deposition. Brasier (1975a) proposed that productivity in carbonate lagoons can be lowered by high organic content, generating the accumulation of toxins and leading to oxygen depletion. Thus, the large amount of Br, a proxy for organic matter, in core BARB1-18-01 could be indicative of oxygen depletion, also explaining the low diversity and quantity of foraminifera within an isolated hypersaline coastal environment (Elbaz-Poulichet et al., 2014; Sabatier et al., 2010a).
Facies 2 (F2) is divided into two alternating subfacies: Facies 2A (F2A) and Facies 2B (F2B) (Figs. 7 and 8). The depths and thicknesses of these subfacies vary between cores, revealing lateral facies variations in the lagoon. Both F2A and F2B consist of sandy and shelly silts. F2A shows higher percentages of fine to coarse sand and shell fragments than F2B. In both subfacies, core BARB1-18-05 always shows higher percentages of coarser grain-size endmembers than core BARB1-18-01. F2A is strongly correlated with the “carbonates” endmembers in both cores, which can be partly explained by many shell fragments (100 µm to several centimeters), indicating a higher-energy environment than during F3 deposition. F2B is more influenced by the “silicates” endmember in core BARB1-18-01 and by the “carbonates” endmember in core BARB1-18-05. The relationship with the silicate endmember in core BARB1-18-01 can again be explained by lower silicate dilution compared to other facies because of the lower sedimentation rate in the area. F2B is mainly composed of a silty Mg-rich calcite matrix with almost undamaged shells of variable size (100 µm to several centimeters) (Fig. 7) and almost no shell fragments, indicating a lower-energy environment than F2A. Moreover, FA2 and F2B both display a fauna dominated by an abundance of individuals of A. angulatus, P. rotunda and many Quinqueloculina species, which were found living inside Codrington Lagoon by Brasier (1975a) in 1975. Furthermore, F2A and F2B display many whole and intact bivalve and gastropod shells, with more abraded shells in F2A. The identified individuals found in F2 are known to live in Codrington Lagoon (Brasier and Donahue, 1985). Thus, F2 records a more carbonate and higher-energy environment (with many broken shells in F2A), in contrast with the very shallow and enclosed lagoon recorded by F3. Such an environment also enables the development of more oxygenated and less toxic conditions for the rich foraminifera fauna to thrive. The presence of whole bivalve and gastropod shells and foraminifera both in F2 and in the lagoon in 1975–1985 CE indicates that the F2 depositional environment was probably similar to the modern lagoon environment (before Hurricane Irma).
The transition from the F3 to the F2 environment seems to be related to relative sea level rise that led to a wider lagoon with a larger fetch allowing wind waves to develop (Fig. 1b). The two 14C ages obtained in F3 are 3277–3451 cal BP (core BARB1-18-01) and 3570–3704 cal BP (core BARB1-18-05). They are in good agreement and correspond to a period of lower sea level, as shown by sea level records by A. palmata corals and microbial mats in Anguilla, Antigua, Barbuda and Martinique (Khan et al., 2017). Khan et al. (2017) showed that the relative sea level (RSL) at 4900 cal BP was m, indicating that during F3 deposition, the RSL was approximately 1 m below the present-day sea level. Such a lower sea level could have great consequences for the shallow Codrington Lagoon, particularly in its northern part, where the average water depth is on the order of 1 m (Fig. 1c). Moreover, a lower sea level implies that the lagoon was probably not, or temporarily, connected with the ocean to the north. Indeed, this shallower sea level could explain the high salt and organic matter content and low diversity and quantity of foraminifera indicative of an isolated coastal environment during F3 deposition.
The deepening recorded by the transition from F3 to F2 could also be explained by subsidence. Along subduction zones, the plate interface (megathrust) can rupture during large earthquakes. Between earthquakes, in the so-called interseismic period, the plate interface, which is the shallower seismogenic portion of the megathrust, is locked. In consequence, stress accumulates at the plate interface and the overriding plate deforms over several decades, centuries or millennia. The area located above the locked portion of the megathrust subsides, whereas that located farther away uplifts. This deformation is suddenly released during an earthquake. When those processes are perfectly elastic, the interseismic and coseismic deformations of the upper plate are strictly of opposite sign and cancel each other (e.g., Savage, 1983). However, long-term topography is building over many seismic cycles in several subduction zones (e.g., Thirumalai et al., 2015; Leclerc and Feuillet, 2019) due to plastic deformation or slip on shallower faults. Barbuda Island is located about 170 km far from the trench, above the seismogenic portion of the plate interface, which undergoes vertical deformation during the seismic cycle of the megathrust (Philibosian et al., 2022). This deformation accumulates at a longer timescale over several seismic cycles to build topography (Weil-Accardo et al., 2022). Microatoll studies along the eastern coasts of the island (Hog Hole) revealed that this island subsided as a response of interseismic strain accumulation over more than 5 decades (1952–2005) at rate of about 5 mm yr−1. The sign of those vertical deformations is compatible with that of the vertical deformation estimated with a GNSS station located few kilometers away to the west but larger by an order of size (0.5 mm yr for a period between 1994 and 2020; van Rijsingen et al., 2022). In this area, Weil-Accardo et al. (2022) studied a set of Quaternary reef terraces and showed that the eastern part of Barbuda has undergone a long-term uplift of 0.05 to 0.1 mm yr−1 over the last 330 kyr. They interpreted this “permanent” deformation as a result of multiple earthquakes. In the western part of the island, however, where Codrington Lagoon is located, there is no evidence of permanent uplift and the island tends to subside over the long term. As in the eastern part, this subsidence was interpreted as a result of deformation over many seismic cycles. Codrington Lagoon may thus have undergone an alternation of coseismic subsidence events and episodes of uplift during the interseismic period. However, these deformations being not perfectly elastic, residual deformation may have accumulated over many seismic cycles, causing the lagoon to subside permanently. The boundary between uplift and subsidence operates over a short distance likely because Barbuda is located just above the downdip limit of the seismogenic part (Weil-Accardo et al., 2022). The abrupt boundary between F3 and F2 in Codrington Lagoon could be the consequence of a sudden subsidence during a major earthquake. Interestingly, Seibert et al. (2019) found a 3500 BP-year-old turbidite and/or homogenite deposit in the Barbuda basin, located offshore east of Barbuda. They concluded that this deposit had been generated by a major earthquake, much larger than all historical events reported in the Lesser Antilles. Given this age is close to the boundary between F3 and F2 in our cores (Fig. 8), this earthquake may have been responsible for the subsidence of Codrington Lagoon, as recorded by a deepening in the lagoon environment. Subsidence of a few meters is thus expected for megathrust events comparable to the Sumatra 2004 and Tohoku 2011 earthquakes (i.e., Ozawa et al., 2011).
Facies 1 (F1) consists of fine sand (main mode at 127 µm) deposited at the top of the six cores (Figs. 7 and 8) and within five thin layers at the bottom of cores BARB1-18-01 and BARB1-18-02. Most of the F1 layers exhibit a sharp basal contact with the underlying facies, and their number decreases with increasing distance from the barrier (Fig. 8). F1 mainly consists of carbonate sand, derived from eroded fragments of marine skeletal material (e.g., corals, mollusks, bivalves and foraminifers) and of shell fragments (100 to 1000 µm). F1 displays the highest foraminifera diversity in cores BARB1-18-01 and BARB1-18-05. This fauna is dominated by autochthonous species, as observed in F2. However, the presence of rare allochthonous marine species indicates a transport from the shoreface into the lagoon during marine incursions. Given that the microtidal regime of Barbuda favors the development of an isolated lagoon (e.g., Boyd et al., 1992), as shown by historical maps and satellite images before Hurricane Irma, the presence of allochthonous species suggests an overwash of the sandy barrier. Consequently, F1 layers are interpreted as overwash deposits related to either hurricanes or tsunamis. The thin F1 layers intercalated within F3 do not present any visible tsunamigenic criteria, such as intraclasts of underlying sediment or mud caps. Given that hurricanes occur much more frequently than tsunamis in Barbuda, we assume that F1 layers intercalated with F3 could record hurricane events. However, it is also possible that one of the F1 layers intercalated in F3 corresponds to the potential 3500 cal BP tsunami event recorded by turbidite and homogenite (Seibert et al., 2019). The F1 layer at the top of cores BARB1-18-01 and BARB1-18-05 displays rather homogeneous 210Pbex values, indicating deposition of the F1 layer triggered by a single rapid event reworking previously deposited material (Fig. 10; Bruel and Sabatier, 2020). Moreover, both 210Pbex profiles exhibit an intermediate value at the base of F1, which may indicate the mixing of sediment from different ages and thus erosion at the base of the F1 deposit. From these observations, we assume that the thick F1 sand layer deposited at the top of the six cores corresponds to a unique high-energy and instantaneous marine flooding event that occurred recently, before core sampling in March 2018 and after the maximum bomb test radionuclide fallout (1963). As no tsunami occurred in Barbuda during this period, the best candidate for this deposit is a hurricane (Fig. 2).
5.2 Hurricane activity since 1750 and morphological evolution of Codrington Lagoon
From 1750 CE to 1973 CE, nine hurricanes, with intensities from 1 to 4, passed within 30 km of Codrington Lagoon (Table 1; NOAA, 2021, historical hurricane tracks). Given no nautical maps (Fig. 3) or historical records indicated the presence of any inlet in the barrier during this period, we assume that none of these hurricanes generated a breach wide and deep enough to evolve toward an inlet through the sandy western barrier. Moreover, satellite images from 1982 to 2017 show a continuous sandy barrier despite the occurrence of four hurricanes of category 1 to 4 that passed within 30 km of Codrington Lagoon (Table 1; NOAA, 2021, Historical Hurricane Tracks; Fig. 4). A washover fan is visible in the December 1999 satellite image to the south of the barrier but did not exist in the previous image of May 1992. Hurricanes Luis (H4) and Bertha (H1), which occurred in 1995 and 1996, respectively, could be good candidates to explain this washover deposit. Potter et al. (2017) mentioned a narrow breach that emplaced across the barrier after Hurricane Luis. Unfortunately, images without clouds are not available between 1995 and 1999 to confirm this observation. However, given that Luis was much stronger than Bertha and Luis made landfall on Barbuda (Table 1; Fig. 2), it is likely that Luis was able to produce the washover observed in 1999. Following category 5 Hurricane Irma (September 2017), two wide inlets, 0.5 and 1.2 km wide, developed and were observed in November 2017 (Fig. 4). The southern inlet closed between March and July 2018 (Fig. 4). In contrast, the northern inlet became larger and was still open in September 2021. Seismic profiling within this inlet in June 2021 shows that a large and thick ebb-tidal delta and a 1 m thick sand sheet developed within the main channel. The permanent opening of a wide inlet 5 years after Hurricane Irma across the sandy barrier supports the idea that this extreme event was exceptional. The permanence of such a wide inlet in a wave-dominated and microtidal environment might seem surprising. Indeed, the stability of tidal inlets can be related to the ratio between the tidal prism (Ω) and the annual net littoral drift (M) (Bruun et al., 1978): the stability of tidal inlets decreases with decreasing . Thus, in a microtidal setting (reduced tidal prism), the tidal inlet tends to be filled by sediment transported by wave-related processes, such as littoral drift and/or cross-shore sand transport. Nevertheless, it can be assumed that along the west coast of Barbuda, waves are very attenuated, leading to small littoral drift, explaining why the inlet remains open. Another hypothesis is related to wind stress on the large surface area of Codrington Lagoon, related to northeast trade winds, that is able to induce strong seaward currents across the inlet.
5.3 Hurricane Irma: an exceptional event
From available data, no wide barrier breach or tidal inlet similar to those developed after Hurricane Irma was documented in Codrington Lagoon. Assuming the Codrington Lagoon barrier remained continuous for more than 250 years, the opening of a wide inlet after Hurricane Irma is an argument for the exceptional character of this event. Inlets opening after major hurricanes have already been reported in other areas (e.g., Sallenger et al., 2006; Chaumillon et al., 2014; Safak et al., 2016). Hurricane Irma also generated beach erosion on many islands such as the islands of Saint Martin and Saint Barthélemy (Rey et al., 2019), Anegada Island (Spiske et al., 2021), and Marco Island in Florida (Harvey et al., 2021).
Moreover, sediment cores show that the upper Codrington Lagoon fill consists of a recent and sharp-based fine-sand sheet (F1) deposited upon the silty lagoon facies (F2). We interpret this sand sheet as recording the devastating passage of Hurricane Irma. This event induced a significant storm surge with a peak water level at 2.4 m, with mean higher high water (MHHW) recorded at a tide gauge on the island. Such exceptionally high sea levels were due to the combined effects of storm surge (resulting from the low-pressure effect and wind stress), wave setup, swash and infragravity waves (e.g., Chaumillon et al., 2017) and allowed overwashing of the 1 to 3 m high sandy barrier. The required sand supply for such a thick and extensive unit would originate from both the breached and eroded sandy barrier and the sandy shoreface. Near the inlet, the sand supply also probably originated from the flood tidal delta. The sandy shoreface origin of this sand supply is supported by the occurrence of allochthonous foraminifera originating from outside the lagoon. Given that this thick sand sheet is unique at the scale of the sediment cores recording a lagoon story longer than 3700 years, it seems that the event responsible for this sand sheet had no equivalent over the last millennia.
Nevertheless, one major limitation of our sedimentological study is related to the absence of an accurate age model and to 14C age inversions. Thus, we cannot rule out that the sedimentation within the lagoon was not continuous and that some hurricanes were not recorded and/or were eroded. For example, the overwash deposit of Hurricane Donna (1960) was probably recorded in Salt Pond, on the eastern part of the island (Knowles, 2008), but no deposit of this age has been identified in the studied cores from Codrington Lagoon. It can be assumed that a deposit of this age has been eroded by Irma. However, the thickness and the lateral extension of the upper F1 sand sheet argue for the unlikeliness of analogous older hurricane records. Another main limitation of our study is related to changes in relative sea level and/or vertical motions during the last 3700 years that can drastically change the sensitivity of the lagoon to overwashes and marine floods. Moreover, the lagoon and barrier morphology has likely changed during the last 3700 years, and we do not have any information about those changes prior to the oldest marine map (1750–1785 CE, Fig. 3). If the spit morphology changed during the last millennia, it would have great consequences for Codrington Lagoon's sensitivity to hurricane sediment record. Other parameters, like the storm track and distance of the storm to the field site, play an important role too (Biguenet et al., 2021). These limitations do not allow us to firmly conclude whether Hurricane Irma was unique at the scale of the last 3700 years.
Even if other events of this intensity occurred in Barbuda Island during the last 3700 years, the consequences of Hurricane Irma remain exceptional in the current climate change context. Thus, the opening of the current lagoon barrier has left the island more exposed to future major hurricanes. This phenomenon has already been observed in a southwest Florida back-barrier lagoon, where the almost complete disappearance of a separate barrier that was once seaward of the current barrier island foreshore occurred during the period that saw the passing of Hurricane Donna (1960 CE). This event was the most significant hurricane to have an impact in the southwest Florida region during modern historical time and has left the island more exposed to major storm events such as Hurricane Irma (Martin and Muller, 2021).
As extreme seasons with category 4–5 hurricanes might be more regular in the future due to current and projected atmospheric and oceanic warming (Pfleiderer et al., 2022), hurricane overwash events and coastal flooding will likely become more prevalent. This increase in major hurricanes associated with the sea level rise exposes a growing number of low-lying coasts and islands to marine submersion, especially barrier islands which are extremely vulnerable landscapes. Thus, it is crucial to understand the coastline geomorphological and sedimentological changes in response to hurricane processes to better inform and prepare coastal communities. More specifically, understanding potential areas of overwash and erosion vulnerability will be crucial for future decisions about coastal resilience and infrastructure planning (Martin and Muller, 2021).
From available satellite images (1982–2021) and old nautical maps (1750–1785 to 1973 CE) prior to Hurricane Irma, the Codrington Lagoon sandy barrier probably remained continuous for at least the last 250 years. No large barrier breaching occurred during the 1990–2000 CE period despite the occurrence of four hurricanes, of category 1 to 4, that passed within 30 km of Codrington Lagoon. However, following category 5 Hurricane Irma, two wide inlets of 0.5 and 1.2 km width developed. The southern inlet has since been closed, but the northern inlet has been enlarged and was still open in September 2021. This inlet allowed the development of a large and thick ebb delta offshore, as shown by seismic profiling. The permanent opening of a wide inlet 5 years after Hurricane Irma across a sandy barrier that has remained continuous for more than 250 years supports the idea that this hurricane was exceptional. Sedimentological, geochemical, microfaunal and chronological analyses conducted on six sediment cores sampled in Codrington Lagoon 7 months after the event showed that Irma led to the deposition of a thick sediment layer in all coring locations, which had no equivalent over the last 3 millennia. Despite limitations related to the absence of an accurate age model and information about the morphological evolution of the lagoon during the last millennia, the sediment record, together with satellite images and old nautical maps, seems to show that Hurricane Irma was exceptional and perhaps unique for the last 3700 years. Our results seem to be in agreement with the occurrence of hurricanes with unprecedented intensities related to present-day global warming. However, given uncertainties related to geomorphological data and sedimentological records, complementary studies need to be pursued in other lagoons of the Caribbean.
All raw data can be provided by the corresponding authors upon request.
The supplement related to this article is available online at: https://doi.org/10.5194/nhess-23-3761-2023-supplement.
MB, EC, PS, FA, TC and NF participated in the fieldwork; MB, AB and EG performed the measurements and analyzed the data; MB wrote the manuscript draft; and EC, PS, FA, TC and NF reviewed and edited the manuscript.
The contact author has declared that none of the authors has any competing interests.
Publisher's note: Copernicus Publications remains neutral with regard to jurisdictional claims made in the text, published maps, institutional affiliations, or any other geographical representation in this paper. While Copernicus Publications makes every effort to include appropriate place names, the final responsibility lies with the authors.
This work is part of the CARQUAKES project (ANR-17-CE03-0006). The authors would like to thank the Ministry of Foreign Affairs, Immigration and Trade of Antigua and Barbuda for permitting core sampling and geophysical surveying in Codrington Lagoon. The authors thank skipper Nicolas Jammes, who was involved in the March 2018 field survey. We thank the GENAVIR captain, officers and crew of R/V ANTEA (Flotte Océanographique Française) and the scientific cruise party, who were involved in the June 2021 field survey. We also thank Louise Cordrie for her valuable support during the 2021 field mission. We thank the Laboratoire Souterrain de Modane (LSM) facilities for the gamma spectrometry measurements. We thank the LMC14 staff (Laboratoire de Mesure du Carbone-14) at the ARTEMIS national facility (LSCE (CNRS-CEA-UVSQ)-IRD-IRSN-MC) for the results obtained with the accelerator mass spectrometry method. The authors would like to thank the EDYTEM laboratory for the X-ray fluorescence analyses and Fayçal Soufi for his involvement in the realization of the thin sections. The authors thank Pierre Vacher and the SYMME Laboratory (Annecy, France) for the authorization to scan core BARB1-18-01 using the X-ray tomography system EasyTom XL 150 from the RX SOLUTION Society.
This work was supported by the CARQUAKES project (ANR-17-CE03-0006) and the Interreg Caraïbes PREST, FEDER European Community (program number CCI 2014TC16RFTN008).
This paper was edited by Ira Didenkulova and reviewed by two anonymous referees.
Abu-Zied, R. H. and Bantan, R. A.: Hypersaline benthic foraminifera from the Shuaiba Lagoon, eastern Red Sea, Saudi Arabia: Their environmental controls and usefulness in sea-level reconstruction, Mar. Micropaleontol., 103, 51–67, https://doi.org/10.1016/j.marmicro.2013.07.005, 2013.
Atwater, B. F., ten Brink, U. S., Cescon, A. L., Feuillet, N., Fuentes, Z., Halley, R. B., Nuñez, C., Reinhardt, E. G., Roger, J. H., Sawai, Y., Spiske, M., Tuttle, M. P., Wei, Y., and Weil-Accardo, J.: Extreme waves in the British Virgin Islands during the last centuries before 1500 CE, Geosphere, 13, 301–368, https://doi.org/10.1130/GES01356.1, 2017.
Bacopoulos, P.: Extreme low and high waters due to a large and powerful tropical cyclone: Hurricane Irma (2017), Nat. Hazards, 98, 939–968, https://doi.org/10.1007/s11069-018-3327-7, 2019.
Bain, A., Faucher, A.-M., Kennedy, L. M., LeBlanc, A. R., Burn, M. J., Boger, R., and Perdikaris, S.: Landscape Transformation During Ceramic Age and Colonial Occupations of Barbuda, West Indies, Environ. Archaeol., 23, 36–46, https://doi.org/10.1080/14614103.2017.1345115, 2018.
Bajard, M., Sabatier, P., David, F., Develle, A.-L., Reyss, J.-L., Fanget, B., Malet, E., Arnaud, D., Augustin, L., Crouzet, C., Poulenard, J., and Arnaud, F.: Erosion record in Lake La Thuile sediments (Prealps, France): Evidence of montane landscape dynamics throughout the Holocene, Holocene, 26, 350–364, https://doi.org/10.1177/0959683615609750, 2016.
Berg, R.: National Hurricane Center tropical cyclone report: Hurricane Jose, NOAA/NWS Rep. AL112017, NOAA, https://www.nhc.noaa.gov/data/tcr/AL122017_Jose.pdf (last access: December 2021), 2018.
Bernard, P. and Lambert, J.: Subduction and seismic hazard in the northern Lesser Antilles: Revision of the historical seismicity, Bull. Seismol. Soc. Am., 78, 1965–1983, 1988.
Bhatia, K. T., Vecchi, G. A., Knutson, T. R., Murakami, H., Kossin, J., Dixon, K. W., and Whitlock, C. E.: Recent increases in tropical cyclone intensification rates, Nat. Commun., 10, 635, https://doi.org/10.1038/s41467-019-08471-z, 2019.
Biguenet, M., Sabatier, P., Chaumillon, E., Chagué, C., Arnaud, F., Jorissen, F., Coulombier, T., Geba, E., Cordrie, L., Vacher, P., Develle, A. L., Chalmin, E., Soufi, F., and Feuillet, N.: A 1600 year-long sedimentary record of tsunamis and hurricanes in the Lesser Antilles (Scrub Island, Anguilla), Sediment. Geol., 412, 105806, https://doi.org/10.1016/j.sedgeo.2020.105806, 2021.
Boger, R., Low, R., and Nelson, P.: Identifying hurricane impacts on Barbuda using citizen science ground observations, drone photography and satellite imagery, Int. Arch. Photogram. Remote Sens. Spat. Inf. Sci., XLII-3/W11, 23–28, https://doi.org/10.5194/isprs-archives-XLII-3-W11-23-2020, 2020.
Boyd, R., Dalrymple, R., and Zaitlin, B. A.: Classification of clastic coastal depositional environments, Sediment. Geol., 80, 139–150, https://doi.org/10.1016/0037-0738(92)90037-R, 1992.
Brasier, M. D.: Ecology of Recent sediment-dwelling and phytal foraminifera from the lagoons of Barbuda, West Indies, J. Foraminif. Res., 5, 42–61, https://doi.org/10.2113/gsjfr.5.1.42, 1975a.
Brasier, M. D.: The ecology and distribution of Recent foraminifera from the reefs and shoals around Barbuda, West Indies, J. Foraminif. Res., 5, 193–210, https://doi.org/10.2113/gsjfr.5.3.193, 1975b.
Brasier, M. D. and Donahue, J.: Barbuda, an emerging reef and lagoon complex on the edge of the Lesser Antilles island arc, J. Geol. Soc. Lond., 142, 1101–1117, 1985.
Breilh, J. F., Chaumillon, E., Bertin, X., and Gravelle, M.: Assessment of static flood modeling techniques: application to contrasting marshes flooded during Xynthia (western France), Nat. Hazards Earth Syst. Sci., 13, 1595–1612, https://doi.org/10.5194/nhess-13-1595-2013, 2013.
Bruel, R. and Sabatier, P.: serac: A R package for ShortlivEd RAdionuclide chronology of recent sediment cores, J. Environ. Radioactiv., 225, 106449, https://doi.org/10.1016/j.jenvrad.2020.106449, 2020.
Bruun, P., Mehta, A. J., and Johnsson, I. G.: Stability of Tidal Inlets. Theory and Engineering, Elsevier Scientific, 506 pp., ISBN 9780444598240, 1978.
Burn, M. J., Holmes, J., Kennedy, L. M., Bain, A., Marshall, J. D., and Perdikaris, S.: A sediment-based reconstruction of Caribbean effective precipitation during the `Little Ice Age' from Freshwater Pond, Barbuda, Holocene, 26, 1237–1247, https://doi.org/10.1177/0959683616638418, 2016.
Cangialosi, J. P., Latto, A. S., and Berg, R.: National Hurricane Center tropical cyclone report: Hurricane Irma, NOAA/NWS Rep. AL112017, NOAA, 111 pp., http://www.nhc.noaa.gov/data/tcr/AL112017_Irma.pdf (last access: December 2021), 2018.
Chagué-Goff, C. and Fyfe, W. S.: Geochemical and petrographical characteristics of a domed bog, Nova Scotia: a modern analogue for temperate coal deposits, Org. Geochem., 24, 141–158, https://doi.org/10.1016/0146-6380(96)00014-9, 1996.
Chagué-Goff, C., Szczuciński, W., and Shinozaki, T.: Applications of geochemistry in tsunami research: A review, Earth-Sci. Rev., 165, 203–244, https://doi.org/10.1016/j.earscirev.2016.12.003, 2017.
Chaumillon, E., Bertin, X., Falchetto, H., Allard, J., Weber, N., Walker, P., Pouvreau, N., and Woppelmann, G.: Multi time-scale evolution of a wide estuary linear sandbank, the Longe de Boyard, on the French Atlantic coast, Mar. Geol., 251, 209–223, https://doi.org/10.1016/j.margeo.2008.02.014, 2008.
Chaumillon, E., Florian, O., Xavier, B., Nathalie, L., and Florian, G.: Control of wave climate and meander dynamics on spit breaching and inlet migration, J. Coast. Res., 70, 109–114, https://doi.org/10.2112/SI70-019.1, 2014.
Chaumillon, E., Bertin, X., Fortunato, A. B., Bajo, M., Schneider, J.-L., Dezileau, L., Walsh, J. P., Michelot, A., Chauveau, E., Créach, A., Hénaff, A., Sauzeau, T., Waeles, B., Gervais, B., Jan, G., Baumann, J., Breilh, J.-F., and Pedreros, R.: Storm-induced marine flooding: Lessons from a multidisciplinary approach, Earth-Sci. Rev., 165, 151–184, https://doi.org/10.1016/j.earscirev.2016.12.005, 2017.
Chester, D. K.: The 1755 Lisbon earthquake, Prog. Phys. Geogr., 25, 363–383, https://doi.org/10.1177/030913330102500304, 2001.
Cordrie, L., Escartin, J., Gailler, A., Feuillet, N., and Heinrich, P.: Simulation of the 2004 tsunami of Les Saintes in Guadeloupe (Lesser Antilles), in: OCEANS 2019 – Marseille, Marseille, France, 1–9, https://doi.org/10.1109/OCEANSE.2019.8867447, 2019.
Cordrie, L., Feuillet, N., Gailler, A., Biguenet, M., Chaumillon, E., and Sabatier, P.: A Megathrust earthquake as source of a Pre-Colombian tsunami in Lesser Antilles: Insight from sediment deposits and tsunami modeling, Earth-Sci. Rev., 228, 104018, https://doi.org/10.1016/j.earscirev.2022.104018, 2022.
Cornée, J.-J., Münch, P., Philippon, M., BouDagher-Fadel, M., Quillévéré, F., Melinte-Dobrinescu, M., Lebrun, J.-F., Gay, A., Meyer, S., Montheil, L., Lallemand, S., Marcaillou, B., Laurencin, M., Legendre, L., Garrocq, C., Boucard, M., Beslier, M.-O., Laigle, M., Schenini, L., Fabre, P.-H., Antoine, P.-O., and Marivaux, L.: Lost islands in the northern Lesser Antilles: possible milestones in the Cenozoic dispersal of terrestrial organisms between South-America and the Greater Antilles, Earth-Sci. Rev., 217, 103617, https://doi.org/10.1016/j.earscirev.2021.103617, 2021.
DeMets, C., Jansma, P. E., Mattioli, G. S., Dixon, T. H., Farina, F., Bilham, R., Calais, E., and Mann, P.: GPS geodetic constraints on Caribbean-North America Plate Motion, Geophys. Res. Lett., 27, 437–440, https://doi.org/10.1029/1999GL005436, 2000.
Denommee, K. C., Bentley, S. J., and Droxler, A. W.: Climatic controls on hurricane patterns: a 1200-y near-annual record from Lighthouse Reef, Belize, Sci. Rep., 4, 3876, https://doi.org/10.1038/srep03876, 2015.
Donnelly, J. P. and Woodruff, J. D.: Intense hurricane activity over the past 5,000 years controlled by El Niño and the West African monsoon, Nature, 447, 465–468, https://doi.org/10.1038/nature05834, 2007.
Donnelly, J. P., Hawkes, A. D., Lane, P., MacDonald, D., Shuman, B. N., Toomey, M. R., van Hengstum, P. J., and Woodruff, J. D.: Climate forcing of unprecedented intense-hurricane activity in the last 2000 years, Earth's Future, 3, 49–65, https://doi.org/10.1002/2014EF000274, 2015.
Dorel, J.: Seismicity and seismic gap in the Lesser Antilles arc and earthquake hazard in Guadeloupe, Geophys. J. Roy. Astron. Soc., 67, 679–695, https://doi.org/10.1111/j.1365-246X.1981.tb06947.x, 1981.
Dunn, G. E.: The Hurricane season of 1960, Mon. Weather Rev., 99–108, 1961.
Elbaz-Poulichet, F., Sabatier, P., Dezileau, L., and Freydier, R.: Sedimentary record of V, U, Mo and Mn in the Pierre-Blanche lagoon (Southern France) – Evidence for a major anoxia event during the Roman period, Holocene, 24, 1384–1392, https://doi.org/10.1177/0959683614540957, 2014.
Engel, M., Oetjen, J., May, S. M., and Brückner, H.: Tsunami deposits of the Caribbean – Towards an improved coastal hazard assessment, Earth-Sci. Rev., 163, 260–296, https://doi.org/10.1016/j.earscirev.2016.10.010, 2016.
Feuillet, N., Beauducel, F., and Tapponnier, P.: Tectonic context of moderate to large historical earthquakes in the Lesser Antilles and mechanical coupling with volcanoes, J. Geophys. Res., 116, B10308, https://doi.org/10.1029/2011JB008443, 2011.
Fuentes, Z., Tuttle, M. P., and Schmidt, W. E.: Sand Scripts of Past Tsunamis in Coastal Ponds of St. Thomas, U.S. Virgin Islands, Seismol. Res. Lett., 88, 1516–1526, https://doi.org/10.1785/0220170038, 2017.
Fullarton, A. and Company: The West Indies. By G. H. Swanston, Edinr. (with) The Most Important Of The Lesser British 835 Islands On An Enlarged Scale. LXI. Engraved. by G. H. Swanston Edinburgh, A. Fullarton & Co. Edinburgh, London, Dublin, David Rumsey Map Collection, http://www.davidrumsey.com/maps3022.html (last access: October 2021), 1872.
Gerritsen, H.: What happened in 1953? The Big Flood in the Netherlands in retrospect, Philos. T. Roy. Soc. A, 363, 1271–1291, https://doi.org/10.1098/rsta.2005.1568, 2005.
Glumac, B. and Curran, H. A.: Documentation of Extensive Root Systems of Thalassia Seagrass Along the Banks of Pigeon Creek, San Salvador Island, Bahamas, in: Geosciences: Faculty Publications, Proceedings of the 16th Symposium on the Geology of the Bahamas and Other Carbonate Regions, Smith College, Northampton, MA, https://scholarworks.smith.edu/geo_facpubs/95 (last access: September 2022), 2016.
Goldberg, E. D.: Geochronology with 210Pb radioactive dating, Radioactive Dating, in: Proceedings of the Symposium on Radioactive Dating Held by the International Atomic Energy Agency in cooperation with the Joint Commission on Applied Radioactivity, 19–23 November 1962, Athens, 121–131, ISBN 9789200306631, ISBN 9200306632, 1963.
Griffis, F. H. (B.): Engineering failures exposed by Hurricane Katrina, Technol. Soc., 29, 189–195, https://doi.org/10.1016/j.techsoc.2007.01.015, 2007.
Harbitz, C. B., Glimsdal, S., Bazin, S., Zamora, N., Løvholt, F., Bungum, H., Smebye, H., Gauer, P., and Kjekstad, O.: Tsunami hazard in the Caribbean: Regional exposure derived from credible worst case scenarios, Cont. Shelf Res., 38, 1–23, https://doi.org/10.1016/j.csr.2012.02.006, 2012.
Harvey, N., Gross, A., Jose, F., Savarese, M., and Missimer, T. M.: Geomorphological impact of Hurricane Irma on Marco Island, Southwest Florida, Nat. Hazards, 106, 1–17, https://doi.org/10.1007/s11069-020-04445-3, 2021.
Heiri, O., Lotter, A. F., and Lemcke, G.: Loss on ignition as a method for estimating organic and carbonate content in sediments: reproducibility and comparability of results, J. Paleolimnol., 25, 101–110, 2001.
Hoggarth, D.: Management Plan for the Marine Parks of Anguilla. Prepared for: Organisation of Eastern Caribbean States Natural Resources Management Unit St Lucia, Department for International Development, 65 pp. https://parkscaribbean.net/wp-content/uploads/2013/11/Anguilla Marine Parks Management Plan (2001).pdf (last access: September 2022), 2001.
Holland, G. and Bruyère, C. L.: Recent intense hurricane response to global climate change, Clim. Dynam., 42, 617–627, https://doi.org/10.1007/s00382-013-1713-0, 2014.
Hough, S. E.: Missing great earthquakes, J. Geophys. Res.-Solid, 118, 1098–1108, https://doi.org/10.1002/jgrb.50083, 2013.
James, P.: Analysis of beach changes in Antigua and Barbuda 1996–2001, Fisheries Division, Ministry of Agriculture, Lands, Fisheries, Antigua and Barbuda, https://docplayer.net/205412792-Analysis-of-beach-changes-antigua-and-barbuda.html (last access: September 2022), 2003.
Javaux, E. J. and Scott, D. B.: Illustration of Modern Benthic Foraminifera from Bermuda and Remarks on Distribution in Other Subtropical/Tropical Areas, Palaeontol. Electron., 6, 1–29, 2003.
Karim, M. F. and Mimura, N.: Impacts of climate change and sea-level rise on cyclonic storm surge floods in Bangladesh, Global Environ. Change, 18, 490–500, https://doi.org/10.1016/j.gloenvcha.2008.05.002, 2008.
Khan, N. S., Ashe, E., Horton, B. P., Dutton, A., Kopp, R. E., Brocard, G., Engelhart, S. E., Hill, D. F., Peltier, W. R., Vane, C. H., and Scatena, F. N.: Drivers of Holocene sea-level change in the Caribbean, Quaternary Sci. Rev., 155, 13–36, https://doi.org/10.1016/j.quascirev.2016.08.032, 2017.
Knowles, J. T.: A 5000 year history o f Caribbean environmental change and hurricane activity reconstructed from coastal lake sediments of the West Indies, LSU Doctoral Dissertations, 4026, https://doi.org/10.31390/gradschool_dissertations.4026, 2008.
Knutson, T., Camargo, S. J., Chan, J. C. L., Emanuel, K., Ho, C.-H., Kossin, J., Mohapatra, M., Satoh, M., Sugi, M., Walsh, K., and Wu, L.: Tropical Cyclones and Climate Change Assessment: Part I: Detection and Attribution, B. Am. Meteorol. Soc., 100, 1987–2007, https://doi.org/10.1175/BAMS-D-18-0189.1, 2019.
Kossin, J. P., Olander, T. L., and Knapp, K. R.: Trend Analysis with a New Global Record of Tropical Cyclone Intensity, J. Climate, 26, 9960–9976, https://doi.org/10.1175/JCLI-D-13-00262.1, 2013.
Kossin, J. P., Knapp, K. R., Olander, T. L., and Velden, C. S.: Global increase in major tropical cyclone exceedance probability over the past four decades, P. Natl. Acad. Sci. USA, 117, 11975–11980, https://doi.org/10.1073/pnas.1920849117, 2020.
Kumar, A., Abouchami, W., Galer, S. J. G., Singh, S. P., Fomba, K. W., Prospero, J. M., and Andreae, M. O.: Seasonal radiogenic isotopic variability of the African dust outflow to the tropical Atlantic Ocean and across to the Caribbean, Earth Planet. Sc. Lett., 487, 94–105, https://doi.org/10.1016/j.epsl.2018.01.025, 2018.
Lander, J. F., Whiteside, L. S., and Lockridge, P. A.: A brief history of tsunamis in the Caribbean Sea, Sci. Tsunami Hazards, 20, 57–94, 2002.
Leclerc, F. E. and Feuillet, N.: Quaternary coral reef complexes as markers of long-term subduction-induced subsidence, Geosphere, 15, 983–1007, https://doi.org/10.1130/GES02069.1, 2019.
Liu, Y., Liu, X., and Sun, Y.: QGrain: An open-source and easy-to-use software for the comprehensive analysis of grain size distributions, Sediment. Geol., 423, 105980, https://doi.org/10.1016/j.sedgeo.2021.105980, 2021.
Malaizé, B., Bertran, P., Carbonel, P., Bonnissent, D., Charlier, K., Galop, D., Imbert, D., Serrand, N., Stouvenot, Ch., and Pujol, C.: Hurricanes and climate in the Caribbean during the past 3700 years BP, Holocene, 21, 911–924, https://doi.org/10.1177/0959683611400198, 2011.
Martin, T. and Muller, J.: The geologic record of Hurricane Irma in a Southwest Florida back-barrier lagoon, Mar. Geol., 441, 106635, https://doi.org/10.1016/j.margeo.2021.106635, 2021.
Mccann, W. R., Dewey, J. W., Murphy, A. J., and Harding, S. T.: A large normal-fault earthquake in the overriding wedge of the Lesser Antilles subduction zone: the earthquake of 8 October 1974, Bull. Seismol. Soc. Am., 72, 2267–2283, 1982.
McCloskey, T. A. and Keller, G.: 5000 year sedimentary record of hurricane strikes on the central coast of Belize, Quatern. Int., 195, 53–68, https://doi.org/10.1016/j.quaint.2008.03.003, 2009.
NGDC/WDS – National Geophysical Data Center/World Data Service: Global Historical Tsunami Database, 900 National Geophysical Data Center, NOAA, https://doi.org/10.7289/V5PN93H7, 2020.
NOAA – National Oceanic and Atmospheric Administration: Historical Hurricane Tracks, https://coast.noaa.gov/hurricanes/#map=4/32/-80 (last access: 15 October 2021), 2021.
Oliver, V. L.: The history of the island of Antigua, one of the Leeward Caribbees in the West Indies, from the first settlement in 1635 to the present time, London, Mitchell and Hughes, 518 pp., https://archive.org/details/historyofislando03oliv/page/n3/mode/2up (last access: December 2021), 1894.
Ozawa, S., Nishimura, T., Suito, H., Kobayashi, T., Tobita, M., and Imakiire, T.: Coseismic and postseismic slip of the 2011 magnitude-9 Tohoku-Oki earthquake, Nature, 475, 373–376, https://doi.org/10.1038/nature10227, 2011.
Pelinovsky, E., Zahibo, N., Dunkley, P., Edmonds, M., Herd, R., Talipova, T., Kozelkov, A., and Nikolkina, I.: Tsunami Generated By the Volcano Eruption On July 12–13, 2003 At Montserrat, Lesser Antilles, Sci. Tsunami Hazards, 22, 44–57, 2004.
Pfleiderer, P., Nath, S., and Schleussner, C.-F.: Extreme Atlantic hurricane seasons made twice as likely by ocean warming, Weather Clim. Dynam., 3, 471–482, https://doi.org/10.5194/wcd-3-471-2022, 2022.
Philibosian, B., Feuillet, N., Weil-Accardo, J., Jacques, E., Guihou, A., Mériaux, A.-S., Anglade, A., Saurel, J.-M., and Deroussi, S.: 20th-century strain accumulation on the Lesser Antilles megathrust based on coral microatolls, Earth Planet. Sc. Lett., 579, 117343, https://doi.org/10.1016/j.epsl.2021.117343, 2022.
Potter, A. E., Chenoweth, S., and Day, M.: Antigua and Barbuda, in: Landscapes and Landforms of the Lesser Antilles, edited by: Allen, C. D., Springer International Publishing, Cham, 99–116, https://doi.org/10.1007/978-3-319-55787-8_8, 2017.
R Core Team: R: A language and environment for statistical computing, R Foundation for Statistical Computing, Vienna, Austria, http://www.r-project.org/index.html (last access: September 2022), 2021.
Reid, H. F. and Taber, S.: The Virgin Islands earthquakes of 1867–1868, Bull. Seismol. Soc. Am., 10, 9–30, 1920.
Reimer, P. J., Austin, W. E. N., Bard, E., Bayliss, A., Blackwell, P. G., Ramsey, C. B., Butzin, M., Cheng, H., Edwards, R. L., Friedrich, M., Grootes, P. M., Guilderson, T. P., Hajdas, I., Heaton, T. J., Hogg, A. G., Hughen, K. A., Kromer, B., Manning, S. W., Muscheler, R., Palmer, J. G., Pearson, C., van der Plicht, J ., Reimer, R. W., Richards, D. A., Scott, E. M., Southon, J. R., Turney, C. S. M., Wacker, L., Adolphi, F., Büntgen, U., Capano, M., Fahrni, S. M., Fogtmann Schulz, A., Friedrich, R., Köhler, P., Kudsk, S., Miyake, F., Olsen, J., Reinig, F., Sakamoto, M., Sookdeo, A., and Talamo, S.: The IntCal20 Northern Hemisphere Radiocarbon Age Calibration Curve (0–55 Cal K BP), Radiocarbon, 62, 725–757, https://doi.org/10.1017/RDC.2020.41, 2020.
Rey, T., Leone, F., Candela, T., Belmadani, A., Palany, P., Krien, Y., Cécé, R., Gherardi, M., Péroche, M., and Zahibo, N.: Coastal Processes and Influence on Damage to Urban Structures during Hurricane Irma (St-Martin & St-Barthélemy, French West Indies), J. Mar. Sci. Eng., 7, 215, https://doi.org/10.3390/jmse7070215, 2019.
Reyss, J.-L., Schmidt, S., Legeleux, F., and Bonté, P.: Large, low background well-type detectors for measurements of environmental radioactivity, Nucl. Instrum. Meth. Phys. Res. Sect. A, 357, 391–397, https://doi.org/10.1016/0168-9002(95)00021-6, 1995.
Richter, T. O., van der Gaast, S., Koster, B., Vaars, A., Gieles, R., de Stigter, H. C., De Haas, H., and van Weering, T. C. E.: The Avaatech XRF Core Scanner: technical description and applications to NE Atlantic sediments, Geol. Soc. Lond. Spec. Publ., 267, 39–50, https://doi.org/10.1144/GSL.SP.2006.267.01.03, 2006.
Robson, G. R.: An earthquake catalog ue for the Eastern Caribbean 1530–1960, Bull. Seismol. Soc. Am., 54, 785–832, https://doi.org/10.1785/BSSA0540020785, 1964.
Roger, J., Baptista, M. A., Sahal, A., Accary, F., Allgeyer, S., and Hébert, H.: The Transoceanic 1755 Lisbon Tsunami in Martinique, Pure Appl. Geophys., 168, 1015–1031, https://doi.org/10.1007/s00024-010-0216-8, 2011.
Sabatier, P., Dezileau, L., Briqueu, L., Colin, C., and Siani, G.: Clay minerals and geochemistry record from northwest Mediterranean coastal lagoon sequence: Implications for paleostorm reconstruction, Sediment. Geol., 228, 205–217, https://doi.org/10.1016/j.sedgeo.2010.04.012, 2010a.
Sabatier, P., Dezileau, L., Barbier, M., Raynal, O., Lofi, J., Briqueu, L., Condomines, M., Bouchette, F., Certain, R., Van Grafenstein, U., Jorda, C., and Blanchemanche, P.: Late-Holocene evolution of a coastal lagoon in the Gulf of Lions (South of France), Bulletin de la Societe Geologique de France, 181, 27–36, https://doi.org/10.2113/gssgfbull.181.1.27, 2010b.
Safak, I., Warner, J. C., and List, J. H.: Barrier island breach evolution: Alongshore transport and bay-ocean pressure gradient interactions, J. Geophys. Res.-Oceans, 121, 8720–8730, https://doi.org/10.1002/2016JC012029, 2016.
Sainte-Claire Deville, C.: Observations sur le tremblement de terre éprouvé à la Guadeloupe le 8 Février 1843, Imprimerie du Gouverneur, Basse-Terre, https://gallica.bnf.fr/ark:/12148/bpt6k625707.r=.langFRen?lang= EN (last access: September 2022), 1843.
Sallenger, A. H., Stockdon, H. F., Fauver, L., Hansen, M., Thompson, D., Wright, C. W., and Lillycrop, J.: Hurricanes 2004: An overview of their characteristics and coastal change, Estuaries and Coasts, Springer, 880–888, https://doi.org/10.1007/BF02798647, 2006.
Savage, J. C.: A dislocation model of strain accumulation and release at a subduction zone, J. Geophys. Res.-Solid, 88, 4984–4996, https://doi.org/10.1029/JB088iB06p04984, 1983.
Schindelin, J., Arganda-Carreras, I., Frise, E., Kaynig, V., Longair, M., Pietzsch, T., Preibisch, S., Rueden, C., Saalfeld, S., Schmid, B., Tinevez, J.-Y., White, D. J., Hartenstein, V., Eliceiri, K., Tomancak, P., and Cardona, A.: Fiji: an open-source platform for biological-image analysis, Nat. Meth., 9, 676–682, https://doi.org/10.1038/nmeth.2019, 2012.
Seibert, C., Feuillet, N., Beck, C., Ducassou, E., Morena, P., Johannes, L., Ratzov, G., Goldfinger, C., Cattaneo, A., and Moreno, E.: Long Term Recurrence of Deeply Ponded Turbidites and Thick Homogenites in the Lesser Antilles Forearc: Imprint of Great Earthquakes [abstract], in: American Geophysical Union, Fall Meeting, 9–13 December 2019, San Francisco, Abstract #OS51C-1503, 2019.
Shepherd, J. B.: Comment on “Subduction and seismic hazard in the Lesser Antilles” by Pascal Bernard and Jerome Lambert, Bull. Seismol. Soc. Am., 82, 1534–1543, 1992.
Simpkin, P. G. and Davis, A.: For seismic profiling in very shallow water, a novel receiver, Sea Technol., 34, 21–28, 1993.
Spiske, M., Pilarczyk, J. E., Mitchell, S., Halley, R. B., and Otai, T.: Coastal erosion and sediment reworking caused by hurricane Irma – implications for storm impact on low-lying tropical islands, Earth Surf. Proc. Land., 47, 891–907, https://doi.org/10.1002/esp.5293, 2021.
Stoddart, D. R., Bryan, G. W., and Gibbs, P. E.: Inland mangroves and water chemistry, Barbuda, West Indies, J. Nat. Hist., 7, 33–46, https://doi.org/10.1080/00222937300770031, 1973.
Takagi, H., Esteban, M., Shibayama, T., Mikami, T., Matsumaru, R., De Leon, M., Thao, N. D., Oyama, T., and Nakamura, R.: Track analysis, simulation, and field survey of the 2013 Typhoon Haiyan storm surge, J. Flood Risk Manage., 10, 42–52, https://doi.org/10.1111/jfr3.12136, 2017.
Thirumalai, K., Taylor, F. W., Shen, C.-C., Lavier, L. L., Frohlich, C., Wallace, L. M., Wu, C.-C., Sun, H., and Papabatu, A. K.: Variable Holocene deformation above a shallow subduction zone extremely close to the trench, Nat. Commun., 6, 7607, https://doi.org/10.1038/ncomms8607, 2015.
Tweedy, M. T.: A history of Barbuda under the Codringtons 1738–1833, Department of History Faculty of Arts, University of Birmingham, Birmingham, https://etheses.bham.ac.uk/id/eprint/5356 (last access: September 2022), 1981.
United Nations Development Programme: Housing Support to Barbuda, United Nations Development Programme, Antigua and Barbuda, https://info.undp.org/docs/pdc/Documents/ATG/Final Housing Support to Barbuda Prodoc.pdf (last access: September 2022), 2019.
van Rijsingen, E., Calais, E., Jolivet, R., De Chabalier, J. B., Robertson, R., Ryan, G. A., and Simythe, S.: Ongoing tectonic subsidence in the Lesser Antilles subduction zone, Geophys. J. Int., 231, 319–326, https://doi.org/10.1093/gji/ggac192, 2022.
Wallace, E. J., Donnelly, J. P., Hengstum, P. J., Wiman, C., Sullivan, R. M., Winkler, T. S., d'Entremont, N. E., Toomey, M., and Albury, N.: Intense Hurricane Activity Over the Past 1500 Years at South Andros Island, The Bahamas, Paleoceanogr. Paleoclimatol., 34, 1761–1783, https://doi.org/10.1029/2019PA003665, 2019.
Watt, S. F. L., Talling, P. J., Vardy, M. E., Heller, V., Hühnerbach, V., Urlaub, M., Sarkar, S., Masson, D. G., Henstock, T. J., Minshull, T. A., Paulatto, M., Le Friant, A., Lebas, E., Berndt, C., Crutchley, G. J., Karstens, J., Stinton, A. J., and Maeno, F.: Combinations of volcanic-flank and seafloor-sediment failure offshore Montserrat, and their implications for tsunami generation, Earth Planet. Sc. Lett., 319–320, 228–240, https://doi.org/10.1016/j.epsl.2011.11.032, 2012.
Weil-Accardo, J., Feuillet, N., Philibosian, B., Guihou, A., Jacques, E., Cabioch, G., Anglade, A., Meriaux, A.-S., and Deschamps, P.: Interaction Between Climate and Tectonics in the Northern Lesser Antilles Inferred From the Last Interglacial Shoreline on Barbuda Island, Geochem. Geophy. Geosy., 23, e2021GC010045, https://doi.org/10.1029/2021GC010045, 2022.
Weltje, G. J., Bloemsma, M. R., Tjallingii, R., Heslop, D., Röhl, U., and Croudace, I. W.: Prediction of Geochemical Composition from XRF Core Scanner Data: A New Multivariate Approach Including Automatic Selection of Calibration Samples and Quantification of Uncertainties, in: Micro-XRF Studies of Sediment Cores, vol. 17, edited by: Croudace, I. W. and Rothwell, R. G., Springer Netherlands, Dordrecht, 507–534, https://doi.org/10.1007/978-94-017-9849-5_21, 2015.
Wigley, P.: The distribution of strontium in limestones on Barbuda, West Indies, Sedimentology, 20, 295–304, https://doi.org/10.1111/j.1365-3091.1973.tb02051.x, 1973.
Winkler, T. S., van Hengstum, P. J., Donnelly, J. P., Wallace, E. J., D'Entremont, N., Hawkes, A. D., Maio, C. V., Sullivan, R. M., and Woodruff, J. D.: Oceanic passage of hurricanes across Cay Sal Bank in The Bahamas over the last 530 years, Mar. Geol., 443, 106653, https://doi.org/10.1016/j.margeo.2021.106653, 2022.
Zahibo, N. and Pelinovsky, E. N.: Evaluation of tsunami risk in the Lesser Antilles, Nat. Hazards Earth Syst. Sci., 1, 221–231, https://doi.org/10.5194/nhess-1-221-2001, 2001.
Zahibo, N., Pelinovsky, E., Yalciner, A. C., Kurkin, A., Koselkov, A., and Zaitsev, A.: The 1867 Virgin Island Tsunami, Nat. Hazards Earth Syst. Sci., 3, 367–376, https://doi.org/10.5194/nhess-3-367-2003, 2003.
Zahibo, N., Pelinovsky, E., Okal, E., Yalçiner, A., Kharif, C., Talipova, T., and Kozelkov, A.: The earthquake and tsunami of November 21, 2004 at Les Saintes, Guadeloupe, Lesser Antilles, Sci. Tsunami Hazards, 23, 25–39, 2005.