the Creative Commons Attribution 4.0 License.
the Creative Commons Attribution 4.0 License.
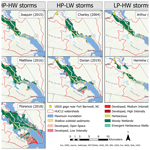
Storm characteristics influence nitrogen removal in an urban estuarine environment
Anne Margaret H. Smiley
Suzanne P. Thompson
Nathan S. Hall
Michael F. Piehler
Sustaining water quality is an important component of coastal resilience. Floodwaters deliver reactive nitrogen (including NOx) to sensitive aquatic systems and can diminish water quality. Coastal habitats in flooded areas can be effective at removing reactive nitrogen through denitrification (DNF). However, less is known about this biogeochemical process in urbanized environments. This study assessed the nitrogen removal capabilities of flooded habitats along an urban estuarine coastline in the upper Neuse River estuary, NC, USA, under two nitrate concentrations (16.8 and 52.3 µM NOx, respectively). We also determined how storm characteristics (e.g., precipitation and wind) affect water column NOx concentrations and consequently DNF by flooded habitats. Continuous flow sediment core incubation experiments quantified gas and nutrient fluxes across the sediment–water interface in marsh, swamp forest, undeveloped open space, stormwater pond, and shallow subtidal sediments. All habitats exhibited net DNF. Additionally, all habitats increased DNF rates under elevated nitrate conditions compared to low nitrate. Structured habitats with high-sediment organic matter had higher nitrogen removal capacity than unstructured, low-sediment organic matter habitats. High-precipitation–high-wind-storm events produced NOx concentrations significantly lower than other types of storms (e.g., low-precipitation–high-wind, high-wind–low-precipitation, low-wind–low-precipitation), which likely results in relatively low DNF rates by flooded habitats and low removal percentages of total dissolved nitrogen loads. These results demonstrate the importance of natural systems to water quality in urbanized coastal areas subject to flooding.
- Article
(4628 KB) - Full-text XML
- BibTeX
- EndNote
Tropical cyclones often cause extensive flooding that can harm ecosystems, damage infrastructure, and disrupt the lives of coastal residents. There is evidence to suggest that anthropogenic climate change has produced conditions (e.g., warmer sea surface temperatures, increased atmospheric moisture) that make these high-magnitude events more likely (Knutson et al., 2013; Min et al., 2011). Since the mid-1990s there has been a shift in storm activity in the USA where tropical cyclones have become slower and rainier and result in catastrophic flooding at higher frequencies than historical averages (Easterling et al., 2017; Kossin, 2018; Kunkel et al., 2010). As climate change continues, some models predict an increase in the most intense storms and up to a 20 % increase in precipitation rates by 2100 (Knutson et al., 2010).
Floodwaters introduce allochthonous materials, including nutrients, to downstream receiving waters. Storm-related upstream discharge typically contains high concentrations of inorganic nitrogen and organic carbon, which constitute up to 80 % of annual loads into receiving waterbodies (Paerl et al., 2020). Estuaries are often nitrogen limited and sensitive to sudden influxes of reactive nitrogen (Howarth and Marino, 2006); therefore floodwaters can trigger water quality degradation by fueling algal blooms (Nixon, 1995) that can disrupt aquatic ecosystems by outcompeting other vegetated habitats for sunlight and nutrients (Wasson et al., 2017). Oftentimes, a product of these blooms is anoxia as heterotrophic remineralization of algal biomass depletes oxygen in the water column, exacerbating negative ecological impacts (Diaz and Roseberg, 1995). Algal blooms are frequently observed following tropical cyclones. For example, one of the largest cyanobacterial blooms in the Okeechobee region has been attributed to Hurricane Irma in 2017 (Hampel et al., 2019), and models showed a strong biological response in Apalachicola Bay following Hurricane Michael in 2018 (D'Sa et al., 2019). Remineralization of algal biomass and terrigenous organic matter by heterotrophic bacteria depletes oxygen in the water column, which can affect health of aquatic organisms and the ecosystem overall (Diaz and Roseberg, 1995).
Watershed urbanization exacerbates water quality degradation in tropical, subtropical, and temperate coastal regions by interfering with hydrologic, geomorphic, and biogeochemical processes (Bowen and Valiela, 2001; Gold et al., 2019, 2021; Lee et al., 2006; Ortiz-Zayas et al., 2006). Population growth has led to increased point source nutrient loading via wastewater effluent into receiving waterways (Carey and Migliaccio, 2009; Naden et al., 2016). Furthermore, impervious surfaces and stormwater pipes streamline flow paths and enhance the export of non-point source anthropogenic nitrogen (Bernhardt et al., 2008). Agricultural landscapes can also deliver nutrients to receiving waterways. In some regions, high densities of agricultural operations substantially increase nutrient concentrations from nitrogen-based fertilizer and animal waste (Duda, 1982; Dupas et al., 2015).
Estuarine habitats are effective at removing terrigenous and anthropogenic nitrogen through a series of biogeochemical reactions (Groffman and Crawford, 2003; Pérez-Villalona et al., 2015; Piehler and Smyth, 2011; Reisinger et al., 2016; Rosenzweig et al., 2018). Denitrification (DNF) is a process by which microbes convert bioavailable forms of nitrogen (nitrate and nitrite) to dinitrogen gas (N2) under anaerobic conditions using carbon as an energy source. DNF is an important process by which reactive nitrogen is naturally and permanently removed from a system. It can be an effective strategy for maintaining water quality during flood conditions that favor DNF, namely, elevated dissolved nitrate and carbon, and anoxia (Adame et al., 2019; Velinsky et al., 2017). Much work has been done to understand DNF by coastal habitats, such as emergent wetlands and oyster reefs (Ensign et al., 2008; Grabowski et al., 2012; Onorevole et al., 2018; Piehler and Smyth, 2011; Velinsky et al., 2017), but much less is known about nitrogen processing in urban landscapes, such as stormwater ponds and lawns/undeveloped open space (UOS), despite their constituting significant area in developed settings. One objective of this study was to quantify nitrogen removal by DNF in flood-prone habitats, both natural and human influenced, including marsh, forested wetland, stormwater pond, undeveloped open space, and shallow subtidal sediments under varied nutrient conditions in Neuse River estuary (NRE), North Carolina (NC).
Storms exhibit unique characteristics which can affect water chemistry differently (Davis et al., 2004; Mallin et al., 2002; Wetz and Paerl, 2008). Some storms produce elevated nutrient concentrations. Sustained winds at high speeds can increase nutrient concentrations by mixing stratified waters and resuspending sediments (Goodrich et al., 1987; Miller et al., 2006; Wengrove et al., 2015). Storms characterized by high precipitation can dilute the nutrients in the water column (Minaudo et al., 2019). Paerl et al. (2020) described the Neuse River estuary (NRE), in eastern North Carolina, as either a “processor” under relatively lower discharge periods where nutrients are able to be partially processed, or a “pipeline” during high-discharge periods where nutrients are delivered to the Albemarle–Pamlico Sound with little processing in the NRE. Therefore, the nitrogen removal capacity of flooded landscapes via DNF is likely influenced by water quality produced during varied storm conditions as well as contact time of floodwaters prior to export from the system. An additional objective of this work was to compare nitrogen loads during multiple storm types to projected nitrogen removal rates by predominant landscape habitats, which were informed by measurements taken as part of the previous objective.
As urban landscapes expand, resulting in losses of natural habitats and wetlands (Aguilera et al., 2020) concomitant with increased anthropogenic nutrient loads, it is essential that we understand the role that both natural and human-influenced landscapes play in removing reactive nitrogen. Additionally, assessing how these habitats perform under a range of nutrient conditions will enable estimation of landscape-scale nitrogen removal capacities during different types of storms. These data will improve our understanding of estuarine nutrient budgets along urbanized coastlines in a new regime of tropical cyclone activity and inform strategic coastal development that maintains ecosystem function to maximize benefits for coastal residents.
2.1 Approach
This study combined laboratory, computational modeling, and geographic information system (GIS) methods to understand landscape-scale DNF capacity during different types of storms. Storm types were defined based on precipitation and wind characteristics. NOx concentrations, NOx loads, and total dissolved oxygen (TDN) loads during those storm events were modeled using weighted regressions. Habitat-specific DNF rates under ambient and elevated nitrate conditions were determined through laboratory experiments. These nitrate treatments represented low and high water column NOx concentrations that are likely associated with different types of storms. Nitrogen removal during these storms was estimated based on experimental DNF values and inundated area of each habitat treatment at maximum inundation. These results were used to draw conclusions about the influence of storm characteristics on water column NOx concentrations and, consequently, biogeochemical processes in flooded landscapes.
2.2 Site description
The upper reaches of the Neuse River estuary (NRE), North Carolina, USA, are influenced by both riverine and coastal hydrologic processes, making them prone to multiple forms of flooding (e.g., fluvial, pluvial, and coastal storm surge). This region also includes both highly urbanized areas and natural ecosystems, affording the opportunity to assess anthropogenic impacts on ecosystem functioning in the context of a built environment. NRE is nutrient sensitive (Boyer et al., 1994; Pinckney et al., 1998; Rudek et al., 1991); primary production is primarily nitrogen limited, and episodic loading events can result in water quality degradation. With headwaters at the urban center of Raleigh and several smaller cities distributed along the river and throughout the watershed, the NRE receives inputs from a 16 000 km2 drainage basin (Christian et al., 1991). Extensive agricultural use paired with rapid urbanization within the watershed makes NRE and similar locations susceptible to water quality degradation during major flood events.
2.3 Storm classification and water quality characteristics
2.3.1 Storm types based on wind and precipitation
Paerl et al. (2018) categorized tropical cyclones that made landfall in North Carolina between 1996 and 2016, based on river discharge at Fort Barnwell and wind speeds at Cape Lookout, NC (NOAA National Data Buoy Center Station CLKN7). The same criteria were used to categorize storms between 2017 and 2019. Storms that resulted in a 7 d mean Neuse River discharge above the 90th percentile of weekly averages (191 m3 s−1) were designated “high precipitation” (HP) events. Those that exhibited a maximum hourly average wind speed above the 90th percentile (14.1 m s−1) between the 12 h prior to and 24 h after landfall were considered “high wind” (HW) events. Storms that produced riverine discharges or wind speeds below these thresholds were considered “low precipitation” (LP) and “low wind” (LW) events, respectively. Storm types were assigned based on both precipitation and wind classifications (Table 1). For example, Hurricane Florence produced both high-precipitation and high-wind conditions and is therefore labeled as a HP–HW storm. Furthermore, storms classified as LP–LW were considered “baseline storm” conditions.
2.3.2 Water quality characteristics during multiple storms
This study compared water quality characteristics during multiple tropical cyclones that affected the North Carolina coast. Average NOx concentrations, NOx loads, and TDN loads on the day of maximum riverine discharge were estimated using Weighted Regressions on Time Discharge and Season (WRTDS) (Hirsch et al., 2010) as described in Paerl et al. (2018) with the following exception. The half window width for the flow term in the model was reduced from the default of 2 ln(flow) increments down to 1 ln(flow) increment to provide greater resolution of flow impacts on concentration and fluxes. This procedure was necessary to capture the observed strong dilution effect of nitrate during extreme, storm-induced flood events. Average concentrations and loads were compared across storm types.
2.4 Nitrogen flux experiment
2.4.1 Sample collection
Sampling for nitrogen flux experiments occurred in October of 2020, timed to capture typical environmental conditions during hurricane season. Sediment cores (6.4 cm diameter with a height of approximately 17 cm) were collected in triplicate from habitats subject to storm flooding including subtidal sediments, stormwater pond, UOS, swamp forest and two marshes – one upstream and one downstream of the outfall from the city of New Bern's wastewater treatment facility (Fig. 1). The two marshes did not exhibit significant differences between mean fluxes and were, therefore, treated as a single habitat treatment.
2.4.2 Dissolved gas and nutrient fluxes across the sediment–water interface
The sediment cores and water were taken to University of North Carolina at Chapel Hill's Institute of Marine Sciences in Morehead City, NC, to conduct dissolved gas and soluble nitrogen flux experiments using methods described by Piehler and Smyth (2011). Sediments and site water were incubated in a temperature-controlled chamber (Bally Inc.) set to in situ water temperature (19 ∘C) in a continuous flow system of water collected from the Neuse River between the two marshes (feedwater). The ambient NOx concentration of the feedwater was 16.8 µM. A peristaltic pump was used to pull feedwater through the water column overlying the sediment cores at a rate of 0.6 L h−1, equating to a turnover time of 5–6 h for water over the sediment in each core. After an overnight equilibration period, water samples were collected from each sediment core outflow for three time points, each 5 h apart. Water pumped directly from feedwater bins was collected at each time point to assess inflow concentrations of dissolved gases and nutrients. After collecting the third time point, the feedwater was enriched with sodium nitrate to a concentration of 52.3 µM, similar to the average NOx concentration modeled for Hurricane Arthur, 47.6 µM, which was the highest observation from our historic storm nutrient data. Following a second overnight equilibration period under nitrate-enriched conditions, three more time points were collected 5 h apart. These two nitrogen treatments are referred to as “low nitrate” (16.8 µM ambient NOx concentration) and “high nitrate” (52.3 µM enriched NOx concentration).
Directly following each water collection (6 total), a membrane inlet mass spectrometer (MIMS; Bay Instruments, Easton, MD) was used to analyze ratios of concentrations of dissolved gases, including N2 : Ar and O2 : Ar, in outflow water samples as well as those collected directly from the feedwater. These measurements were used to calculate net N2 and O2 fluxes across the sediment–water interface. In this study, net N2 fluxes are referred to as DNF. However, there are multiple processes that influence net N2 flux, including DNF, nitrogen fixation, and anammox. O2 fluxes are multiplied by −1 to obtain sediment oxygen demand (SOD). At time points 2 and 5, additional 50 mL water samples were collected to measure nutrient fluxes based on core inflow and outflow concentrations. Samples were filtered through 0.7 µm Whatman GF/F filters and stored at −18 ∘C prior to analysis with a Lachat Quick-chem 8000 (Lachat Instruments, Milwaukee, WI, USA). Nutrient analytes included dissolved inorganic nitrogen species (DIN): nitrate + nitrite (NOx) and ammonium (NH) as well as total dissolved nitrogen (TDN) allowing calculation of dissolved organic nitrogen (DON) by difference. TDN measurements included NOx, NH, and DON. At the end of the experiment, water was drained from the cores and sediment samples were collected from the top 2 cm to determine percent sediment organic material (SOM) based on loss on ignition (Byers et al., 1978; Smyth et al., 2015).
2.5 Spatial data acquisition
2.5.1 Habitat treatments
Distributions and total surface area of sampled habitats were determined using a variety of spatial datasets. Marshes, swamp forests, and UOS were delineated using the National Land Cover Dataset (NLCD), a 30 m raster dataset obtained from remotely sensed Landsat imagery. Land cover classified as emergent and forested wetlands were considered marsh and swamp forest, respectively. Area of UOS was calculated by combining the herbaceous classification and weighted estimates from the various development categories (e.g., open space, low–high intensity). Pixels considered “developed, open space” in the NLCD are defined as those comprised of less than 20 % constructed surfaces. The remaining 80 % of the pixel area was considered UOS, colloquially referred to as lawns and grasses. Low-, medium-, and high-intensity developed pixels were considered 51 %, 21 %, and 0 % UOS, respectively. NLCD datasets have been updated roughly in 2- to 3-year intervals. This work references datasets from multiple years, including 2004, 2016, and 2019.
Shallow subtidal sediments were identified using NOAA's Continuously Updated Digital Elevation Model (CUDEM) and were defined as those within 1 m of the surface. Stormwater infrastructure data were obtained from the city of New Bern and include managed stormwater ponds. ArcGIS Pro 2.8.7 was used to extract NLCD and CUDEM data from 2 HUC12 watersheds in the upper NRE.
2.5.2 Inundation extents for multiple storms
Flood footprints that delineated inundated landscapes for seven selected storms were generated from the Advanced Circulation (ADCIRC) model and acquired from the Coastal Emergency Risks Assessment website (https://cera.coastalrisk.live/). This analysis includes hurricanes Charley (14 August 2004), Arthur (4 July 2014), Joaquin (3 October 2015), Hermine (2 September 2016), Matthew (8 October 2016), Florence (14 September 2018), and Dorian (6 September 2019). Flood footprints for LP–LW storms were not available, so these types of baseline storm events were not considered.
2.6 Calculations and statistical analysis
2.6.1 Dissolved gas and nutrient fluxes
Fluxes of nutrient and dissolved gases across the sediment–water interface were calculated by multiplying the difference between inflow and outflow concentrations by the peristaltic pump/flow rate and dividing by the surface area of the sediment core as in Eq. (1).
Denitrification efficiency was calculated by dividing N2–N fluxes by the total inorganic nitrogen flux out of the sediments, then multiplying by 100, following Eq. (2).
Mean fluxes for dissolved gases and nutrients were compared using Kruskal–Wallis and post hoc Dunn tests to identify differences across landscape treatments and between nutrient treatments. Linear regressions were performed to compare variations in N2–N fluxes to variations in SOD under ambient and nitrate-enriched conditions. Additional linear regressions were used to compare variability in DNF to SOM under both low-nitrate and high-nitrate conditions. All statistical tests were done using R version 4.1.1 (R Core Team, 2021) and were considered significant when p < 0.05.
2.6.2 Nitrogen concentrations and loads during storms
Mean NOx concentrations, NOx loads, and TDN loads on the day of maximum riverine discharge were compared across storm types using Kruskal-Wallis and post hoc Dunn tests. Differences between mean NOx concentrations were used to draw comparisons between experimental nitrate treatments to environmental NOx concentrations during different types of storms. Mean load values were compared to estimate nitrogen removal by flooded landscapes during multiple storms.
2.6.3 Nitrogen removal by the flooded landscape
Nitrogen removal was estimated for seven selected storms with available flood footprints. Tools in ArcGIS Pro were used to extract land cover data from each storm's flood footprint within the two HUC12 watersheds in the upper NRE. The 2004 NLCD dataset was used to estimate the inundated area during Hurricane Charley; the 2016 dataset was used to estimate inundated area for hurricanes Arthur, Joaquin, Hermine, and Matthew; and the 2019 dataset was used for hurricanes Florence and Dorian. For each habitat type, inundated surface areas and mean DNF rates obtained from the nitrogen flux experiments were multiplied to estimate habitat-specific N removal rates, as in Eq. (3). Areas of shallow subtidal sediments were assumed to have remained constant over this time range. The 30 m resolution of the NLCD is too coarse to capture most stormwater ponds in this region; thus this habitat treatment was not considered in this analysis. Removal rates under both high- and low-nitrate conditions were calculated.
3.1 Storm characteristics
HP–HW storms yielded a mean NOx concentration of 11.7 with a standard error of 2.50 µM (n=11) on the day of maximum riverine discharge, which was lower than mean concentrations for the other three storm types (HP–LW: 25.2 ± 6.00 (n=4); LP–HW: 29.3 ± 2.23 (n=14); LP–LW: 24.5 ± 2.16 (n=8); Fig. 2). The low-nitrate experimental treatment (16.8 µM) was considered more representative of the HP–HW events, while the high-nitrate treatment (52.3 µM) was considered more analogous to the other three storm types. Mean NOx loads on the day of maximum discharge during low-precipitation storms were significantly lower than loads during high-precipitation storms (Fig. 2). TDN loads during HP–HW events were higher than low-precipitation storms, and LP–LW events produced loads lower than high-precipitation events. There were no significant differences in mean TDN load between HP–LW and LP–HW storms (Fig. 2). The fraction of NOx in TDN loads also differed across storm types. The proportion on NOx was higher during low-precipitation events (74.2 % in LP–HW storms and 44.8 % in LP–LW storms) than high-precipitation events (16.5 % in HP–HW storms and 20.81 % in HP–LW storms).
3.2 Nitrogen fluxes across the sediment–water interface
Under the low-nitrate conditions, all habitats exhibited net DNF (Fig. 3a). N2–N fluxes in marsh sediments were significantly higher than shallow subtidal, swamp forest, and stormwater pond sediments (Fig. 3a). Following nitrate enrichment, all landscapes experienced a significant increase in DNF rates compared to respective fluxes under low-nitrate conditions (Fig. 3a). Under high-nitrate conditions, marsh and stormwater pond cores produced significantly higher DNF rates than both UOS and subtidal sediments. Swamp forest cores also exhibited higher rates than the subtidal cores (Fig. 3a).
NOx flux was near 0 µmol for each habitat under low-nitrate conditions (Fig. 3b), with no significant differences between means. Following the nitrate addition, each habitat exhibited a significant decrease in fluxes (Fig. 3b). High NOx fluxes were negative for all habitat treatments, indicating NOx moving from the water column into the sediments; thus, each habitat acted as a NOx sink post-enrichment. NOx fluxes were not different between habitats. fluxes were an order of magnitude lower than N2 and NOx fluxes (Fig. 3c). Some fluxes were positive, while others were negative, although no significant differences across habitat or nitrate treatments were evident.
Average DNF efficiencies for all habitats and nitrate treatments were above 70 % (Fig. 3d). Under low-nitrate conditions, UOS was the most efficient habitat, significantly more efficient than marsh, stormwater pond, and shallow subtidal sediments. Following nutrient enrichment, marsh, stormwater pond, and shallow subtidal sediments showed a significant increase, and all habitat treatments nearly reached 100 % DNF efficiency.
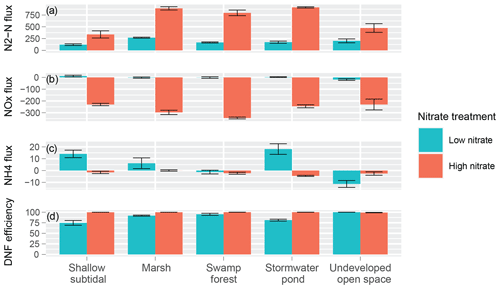
Figure 3Fluxes (µmol ) across the sediment–water interface for multiple nitrogen species, including (a) N2–N, (b) NOx, and (c) NH4, as well as (d) DNF efficiencies (%). Positive fluxes indicate movement from the sediments to the overlying water column.
A linear regression analysis revealed that under low-nitrate conditions, variability in SOD explains approximately 70 % of the variability in DNF (Fig. 4). No significant relationship was evident between DNF and SOD under high-nitrate conditions. There was a significant relationship between SOM and high-nitrate DNF rates, where variability in SOM accounted for roughly 62 % of the variability in N2–N flux.
3.3 Nitrogen removal during storms
Nitrogen removal was calculated for seven named storms with available flood footprints (of three precipitation/wind types, Table 1). Habitat-specific DNF rates were extrapolated across inundated surface areas for each habitat treatment using DNF rates from both low- and high-nitrate treatments. Flood footprints were not available for any LP–LW storms, so these baseline storm events were not included in this analysis. Removal rates calculated using low-nitrate DNF rates ranged between 15.3 and 65.5 kg N h−1. Removal rates in elevated nitrate treatments ranged between 58.4 and 257 kg N h−1. Rates from the low-nitrate treatment were considered more representative of HP–HW events, and rates from the elevated nitrate treatment were considered more representative of HP–LW and LP–HW events. The elevated nitrate treatment was representative of the highest modeled nitrate concentrations in our historic storm nutrient data. Peak nitrate concentration in the experiment was higher than the historic storm nitrate data, resulting in higher modeled removal rates for historic storms.
These removal rates were used to calculate the percent of TDN and NOx loads that were removed by habitats within floodplains for the seven selected storms. Under low-nitrate conditions, the percentage of TDN load removed ranged from 1.15 to 5.95; under high-nitrate conditions, they ranged from 4.81 to 24.6. Regarding NOx loads, under low-nitrate conditions, percent removed ranged from 5.71 to 21.6. Under high-nitrate conditions, they ranged from 21.8 to 84.6.
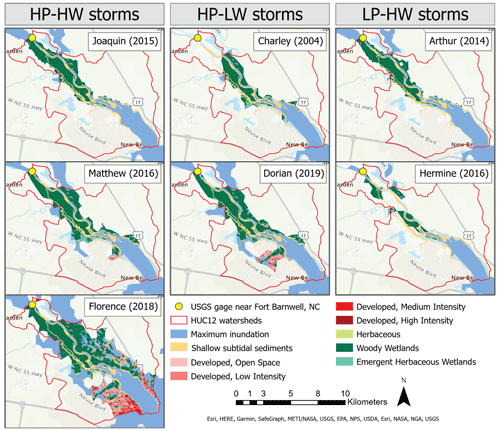
Figure 5NLCD land cover classifications within HUC12 watershed boundaries and floodplain footprints for multiple storms affecting the Neuse River estuary. Habitat treatments are derived from these land cover classifications.
Floodplain footprints varied in size, with each storm inundating different proportions of each habitat (Fig. 5). In each case, swamp forests were the most abundant inundated habitat in the floodplain, comprising between 44.9 % and 66.2 % of the flooded habitat area. Their abundance paired with their relatively high DNF rates is reflected in their high contribution to nitrogen removal overall (Fig. 6). Swamp forests were likely responsible for removing between 51.6 % and 70.1 % of nitrogen removed
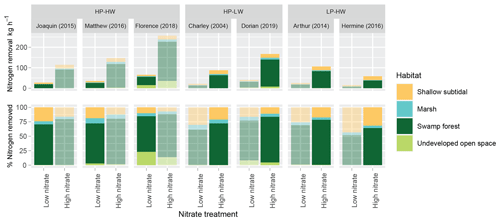
Figure 6Projected nitrogen removal rates (top) and percent contribution (bottom) by each habitat under low- and high-nitrate conditions during multiple storms. Higher color intensity indicates applicable nitrogen level based on storm type.
via DNF by all habitats under low-nitrate conditions and between 64.0 % and 79.4 % under high-nitrate conditions. Shallow subtidal sediments also consistently comprised a large proportion of flooded habitat area, between 12.4 % and 52.2 %. Although DNF rates in this habitat were relatively low, their abundance led to large contributions to nitrogen removal during storms, between 10.1 % and 43.3 % under low-nitrate conditions and between 7.27 % and 31.6 % under high-nitrate conditions. Marshes are relatively sparse in this region of the NRE and contributed a small percentage of nitrogen removal. UOS also consistently made up a small portion of inundated landscapes, though the contribution of UOS seems to have increased in the most recent storms: Matthew, Florence, and Dorian (2.33 %, 13.0 %, and 6.57 % of the flooded habitat area, respectively).
Another consideration is the effect that NOx concentrations have on the relative contribution to overall nitrogen removal by each habitat. Not all habitats responded the same to elevated nitrate conditions. For example, UOS sediments did not increase DNF rates in response to elevated NOx concentrations to the same degree as other habitats, such as swamp forest, do (Fig. 2). Therefore, under high-nitrate conditions, UOS contributed a smaller proportion to nitrogen removal than under low-nitrate conditions (Fig. 6). The same was observed with subtidal sediments. Swamp forests, on the other hand, reliably increased the proportion of their contribution under high-nitrate conditions.
Table 2Summary of NOx concentrations (µM) and average nitrogen loads (NOx and TDN; kg h−1) on the day of maximum discharge and percent of load removed by habitats under low- and high-nitrate conditions. Asterisks indicate the more representative percentage based on water column nitrate concentrations during different storm types.
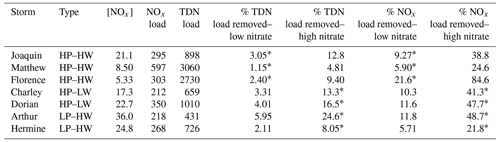
The results of this study shed light on nitrogen removal capacities of multiple flood-prone natural and human-influenced habitats. Few studies have investigated nitrogen removal by habitats in the context of a built environment (Denman et al., 2016; Groffman and Crawford, 2003; Reisinger et al., 2016; Rosenzweig et al., 2018), and even fewer studies have quantified nitrogen processing by urban landscapes, such as stormwater ponds and lawns (Gold et al., 2017; Hohman et al., 2021; Raciti et al., 2011). As coastlines continue urbanizing, these features are increasingly abundant and comprise an important piece of coastal nutrient budgets. Additionally, this study explores how characteristics of storms can influence nitrogen removal capacity by coastal landscapes. This information is important in the context of climate change and the projected increase in high-precipitation storms.
4.1 Nitrogen removal by habitat treatments
Positive N2–N fluxes indicate that all habitats are capable of permanently removing nitrogen under high- and low-nitrate conditions, although DNF rates varied across habitats and between nitrate treatments for some habitats. Under low-nitrate conditions, marsh sediments produced the highest DNF rates and shallow subtidal sediments produced the lowest. Differences in DNF rates between structured habitats, like marshes, and unstructured habitats, like subtidal sediments, have been documented in previous studies (Piehler and Smyth, 2011). These differences have been attributed predominantly to the availability of organic carbon. Carbon availability may explain differences between marshes and other habitats as well. Swamp forests are structured habitats, like marshes; however, the forest sediments exhibited DNF rates that were lower than the marsh sediments under low-nitrate conditions. The quality of carbon affects DNF (Hill and Cardaci, 2004; Seitzinger, 1994), and the molecular structure of sediment organic matter in marshes is simpler and more readily decomposed than sediment organic matter in mangrove forests (Sun et al., 2019). Thus, sediment organic matter in the inundated forests in the present study may have been more recalcitrant than the organic matter in marshes.
There was not a significant difference between DNF rates in marshes and UOS under low-nitrate conditions. The few studies that have examined nitrogen processing in urban UOS have shown that grasses can exhibit high DNF activity (Groffman et al., 1991) but is spatially and temporally heterogenous (McPhillips et al., 2016; Raciti et al., 2011). Multiple factors can influence nitrogen processing, including fertilizer application, soil saturation, species of grass, and temperature (Mancino et al., 1988; Wang et al., 2013). When soils are saturated and temperatures are high, turfgrass sediments can remove up to 93 % of applied nitrogen via DNF (Mancino et al., 1988). Experimental conditions in the present study were similar to those in Mancino et al. (1988), and results suggest that UOS are important for system nitrogen budget under low-nitrate conditions.
UOS sediments were unique in that they exhibited 100 % DNF efficiency under low-nitrate conditions. This could be explained, in part, by organic carbon availability. Eyre and Ferguson (2009) describe critical carbon loading range to maximize DNF efficiency. High DNF efficiency exhibited by UOS sediments under low-nitrate conditions may be due to the presence of labile organic carbon in the soils that falls within a critical range; extremely high organic carbon may create a thick anoxic layer that is unsuitable for aerobic nitrifying bacteria that produce nitrate used in DNF. In contrast, organic carbon that is too low may promote an aerobic layer unsuitable for the anaerobic denitrifying bacteria. Additionally, grasses have been shown to be extremely efficient at nutrient assimilation (Petrovic, 1990). It is plausible that much of the inorganic nitrogen that would have otherwise fluxed out of the sediments was integrated into biomass.
Like grasses, stormwater ponds are prolific features of developed landscapes, and yet few studies have examined their nitrogen processing capabilities. Low nitrate–DNF rates exhibited by the stormwater pond sampled in our study were low relative to the marsh but were higher than those from other studies (Gold et al., 2017, 2021). The pond sampled in this study is part of a restored wetland that feeds a tributary creek of the Neuse River; this hydrological connectivity may partly explain the high DNF rates in this pond relative to others. Gold et al. (2017) describe alternative, less environmentally desirable processes in traditional stormwater ponds that are likely stimulated by poor circulation, thermal stratification, and anoxia in bottom waters. These processes include dissimilatory nitrate reduction to ammonium (DNRA) that could increase the supply of inorganic nitrogen to the system, as well as increased phosphate fluxes from the sediments to the bottom waters resulting in nitrogen limitation. In tandem, these processes may trigger algal blooms that degrade water quality. Flooding from the Neuse River could increase circulation to reduce stratification and prolonged anoxia. Additionally, the natural vegetation that surrounds the stormwater pond could provide a source of organic carbon to the sediments, much higher than a typical urban stormwater pond (Blaszczak et al., 2018; Hohman et al., 2021).
Following nitrate enrichment, all habitats exhibited significant increases in DNF. This type of biogeochemical response has been observed in other studies (Gold et al., 2021; Seitzinger, 1994; Smyth et al., 2015) with marsh, swamp forest, and stormwater pond sediments producing the highest rates. These habitats also exhibited the highest percentages of SOM. It is possible that these high SOM environments were nitrate limited and organic carbon was in excess; therefore, under low-nitrate conditions there is a portion of SOM that was not used in the DNF process. This is supported by the weak linear relationship between SOM and DNF under low-nitrate conditions. The significant positive linear relationship between SOM and DNF under high-nitrate conditions supports that an external source of nitrate may have alleviated this limitation with abundant SOM readily available. A similar phenomenon was observed in the study of Arango et al. (2007) study examining denitrification in streams in the Midwest of the USA. To summarize, habitats showed increased DNF capacity in response to elevated NOx concentrations, with high SOM environments playing the most important roles in nitrogen removal when water column NOx concentrations were high.
The significant positive linear relationship between DNF and SOD at low-nitrate concentrations is consistent with results obtained by Piehler and Smyth (2011) and Seitzinger et al. (2006), suggesting that DNF is tightly coupled with nitrification; the nitrate fueling DNF is produced in situ. Under high-nitrate conditions, the relationship between SOD and DNF was no longer significant. This weak relationship, as well as negative NOx fluxes post-nitrate enrichment, could point to an increased importance of direct DNF, where overlying water supplies nitrate for DNF in the sediments. However, NOx fluxes into the sediments do not completely account for the increased N2–N fluxes out of the sediments under high-nitrate conditions. This underscores the contribution of additional processes, such as coupled nitrification–DNF and anammox.
4.2 Nitrogen removal during different types of storms
Comparing multiple storms that have affected North Carolina's coast revealed that HP–HW storms resulted in water column NOx concentrations that were significantly lower than HP–LW, LP–HW, and LP–LW storms. During HP–HW events, riverine discharge and wind-driven storm surge may dilute NOx concentrations. This dilution effect may also explain the reduced proportion of NOx in TDN loads during high-precipitation events relative to low-precipitation events (Minaudo et al., 2019). NOx concentrations can significantly alter biogeochemical processes, namely DNF. Therefore, the effectiveness of nitrogen removal by the coastal landscape may depend on the type of storm impacting the region. Results from this work suggest that flooded landscapes are permanently removing water column nitrogen through direct DNF at higher rates during HP–LW, LP–HW, and LP–LW storms, compared to HP–HW storms, when NOx concentrations are relatively low and coupled nitrification–DNF is likely the dominant process.
These results largely reinforce the idea put forth by Paerl et al. (2018) where the Neuse River acts as a “pipeline”, delivering nitrogen to Pamlico Sound during these rainier events, as opposed to a “processor” during drier events. Though NOx concentrations during HP–HW storms were relatively low, the high volume of water during wetter storms delivers larger loads of TDN and nitrate to the estuary compared to drier storms. Reduced nitrogen removal capacity of the coastal landscape during these flood events paired with increases in nitrogen loads has implications for greater nitrogen export into Pamlico Sound, especially as climate changes increase the magnitude and frequency of these rainier storms (Easterling et al., 2017; Knutson et al., 2010; Paerl et al., 2019).
Potentially exacerbating this threat to water quality is development within the Neuse River watershed. This study sheds light on how human impacts on the landscape influence distributions of nitrogen sinks as anthropogenic nitrogen sources increase. As urban and suburban landscapes expand, UOS will become more abundant and their role in regulating water quality will grow. These results suggest that under low-nitrate conditions, DNF rates in UOS sediments are comparable to marshes and they will play an important role during flooding from HP–HW storms and other low-nitrate scenarios. They likely will not play as large a role during other types of storms when water column nitrate concentrations are relatively high.
Just as developed landscapes are expanding within watersheds and along coastlines, it seems as though floodplains are infringing on these built environments (Sebastian et al., 2019; Wang et al., 2017; Wobus et al., 2017). Some experts cite a regime shift in tropical cyclone activity where annual exceedance percentages historically used to delineate floodplains (e.g., 100- and 500-year floodplains) are no longer representative of the current conditions (Paerl et al., 2019). When assessing land cover within each storm's floodplain and the nitrogen removal by each habitat, UOS played an increasingly important role during the most recent storms (Matthew, Florence, and Dorian). It is possible that their growing abundance within storm floodplains and their increased contribution to nitrogen removal informs a trend where floodplain boundaries are encroaching further inland (Knutson et al., 2013; Min et al., 2011).
In the upper NRE, when NOx concentrations are high, more natural landscapes – including the hydrologically and ecologically connected stormwater pond sampled in this study – produced the highest DNF rates. However, the limited areal extent of marshes and stormwater ponds within each storm's floodplain rendered them incapable of removing substantial nitrogen in this region. Swamp forests, on the other hand, appear to play an important role in maintaining water quality during storms. They were consistently the most extensive habitat within the storms' floodplains and, as such, made the largest contribution to nitrogen removal under both low- and high-nitrate conditions. Therefore, swamp forests appear to be essential for regulating water quality in the NRE during storms of varying characteristics.
The results of this study provide information about nitrogen removal capacities by multiple natural habitats and urban landscape features in a flood-prone, developed, estuarine environment. All habitats removed nitrogen under low-nitrate conditions and increased their nitrogen removal capacity in response to added nitrate. Flooded UOS can play an important role in regulating nitrogen when water column concentrations are relatively low (e.g., during rainier and windier storms). When water column nitrate concentrations are high, more “natural” structured habitats, including marshes and swamp forest along with a somewhat unique stormwater/wetland pond, were more effective at removing nitrogen than shallow subtidal sediments and UOS. These differences in processing suggest that abundance and spatial distributions of these habitats within a floodplain can influence overall nitrogen removal capacity at the watershed scale.
Water column nutrient concentrations produced by different types of storms likely influence biogeochemical processing by flooded habitats and subsequent nitrogen export downstream and into Pamlico Sound. Results of this study suggest that flooded landscapes may be less effective at removing reactive nitrogen during HP–HW storms compared to other storm types. Low water column NOx concentrations produced during HP–HW events likely result in relatively low DNF rates. DNF rates are likely higher during storm events that produce relatively high water column NOx concentrations, such as HP–LW, LP–HW, and LP–LW storms. Swamp forests are the most extensive of the habitats in this study area and play an important role in removing nitrogen and regulating water quality, regardless of storm characteristics. Management strategies should continue prioritizing swamp forest conservation in this region, as in North Carolina's Riparian Buffer Protection Program.
As coastlines and watersheds become more developed and climate change increases the frequency and magnitude of storms and major flooding events, the export of both anthropogenic and terrigenous nitrogen will likely increase. Understanding nitrogen removal capabilities and limitations of flooded natural coastal habitats as well as those urban landscapes that will become more and more prevalent will enable us to make informed management decisions to benefit the integrity of our coastal waters.
Land cover data, watershed boundaries, and flood footprint data are open source and accessible online (https://doi.org/10.5066/P9KZCM54, Dewitz and U.S. Geological Survey, 2021; https://hydro.nationalmap.gov/arcgis/rest/services/wbd/MapServer/6, U.S. Geological Survey, 2019; https://cera.coastalrisk.live/, Coastal Emergency Risks Assessment, 2022). Wind and riverine discharge datasets are also open source and accessible online (https://www.ndbc.noaa.gov/station_page.php?station=clkn7, NOAA, National Data Buoy Center, 2023; https://waterdata.usgs.gov/monitoring-location/02091814/#parameterCode=00065&period=P7D&showMedian=true, U.S. Geological Survey, 2023). Data collected during the sediment core incubation experiment are available upon request.
AMHS, SPT, and MFP conceptualized the framework for this research. AMHS conducted a literature review, analyzed data, developed figures, and led writing efforts with contributions from SPT, MFP, and NSH. NSH conducted weighted regression on time, season, and discharge analysis.
The contact author has declared that none of the authors has any competing interests.
Publisher’s note: Copernicus Publications remains neutral with regard to jurisdictional claims made in the text, published maps, institutional affiliations, or any other geographical representation in this paper. While Copernicus Publications makes every effort to include appropriate place names, the final responsibility lies with the authors.
This research was supported by funding from the National Science Foundation Growing Convergent Research (award no. 2021086) and the North Carolina Collaboratory award. We would like to thank Piehler lab members at the UNC Institute of Marine Sciences, Mollie Yacano and Chelsea Brown, for their assistance with sample collection and laboratory experiments. We also thank the anonymous reviewers for their helpful comments.
This research has been supported by the National Science Foundation (grant no. 2021086), the National Science Foundation Growing Convergent Research (award no. 2021086), and the North Carolina Collaboratory (project collab_75).
This paper was edited by Philip Ward and reviewed by two anonymous referees.
Adame, M. F., Roberts, M. E., Hamilton, D. P., Ndehedehe, C. E., Reis, V., Lu, J., Griffiths, M., Curwen, G., and Ronan, M.: Tropical Coastal Wetlands Ameliorate Nitrogen Export During Floods, Front. Mar. Sci., 6, 671, https://doi.org/10.3389/fmars.2019.00671, 2019.
Aguilera, M. A., Tapia, J., Gallardo, C., Nunez, P., and Varas-Belemmi, K.: Loss of coastal ecosystem spatial connectivity and services by urbanization: Natural-to-urban integration for bay management, J. Environ. Manage., 276, 111297, https://doi.org/10.1016/j.jenvman.2020.111297, 2020.
Arango, C. P., Tank, J. L., Schaller, J. L., Royer, T. V., Bernot, M. J., and David, M. B.: Benthic organic carbon influences denitrification in streams with high nitrate concentrations, Freshwater Biol., 52, 1210–1222, https://doi.org/10.1111/j.1365-2427.2007.01758.x, 2007.
Bernhardt, E. S., Band, L. E., Walsh, C. J., and Berke, P. E.: Understanding, managing, and minimizing urban impacts on surface water nitrogen loading, Ann. NY Acad. Sci., 1134, 61–96, https://doi.org/10.1196/annals.1439.014, 2008.
Blaszczak, J. R., Steele, M. K., Badgley, B. D., Heffernan, J. B., Hobbie, S. E., Morse, J. L., Rivers, E. N., Hall, S. J., Neill, C., Pataki, D. E., Groffman, P. M., and Bernhardt, E. S.: Sediment chemistry of urban stormwater ponds and controls on denitrification, Ecosphere, 9, e02318, https://doi.org/10.1002/ecs2.2318, 2018.
Bowen, J. L. and Valiela, I.: The ecological effects of urbanization of coastal watersheds: historical increases in nitrogen loads and eutrophication of Waquoit Bay estuaries, Can. J. Fish. Aquat., 58, 1489–1500, https://doi.org/10.1139/cjfas-58-8-1489, 2001.
Boyer, J. N., Stanley, D. W., and Christian, R. R.: Dynamics of NH4+ and NO uptake in the water column of the Neuse River Estuary, North Carolina, Estuaries, 17, 361–371, 1994.
Byers, S. C., Mills, E. L., and Stewart, P. L.: A comparison of methods of determining organic carbon in marine sediments, with suggestions for a standard method, Hydrobiologia, 58, 43–47, 1978.
Carey, R. O. and Migliaccio, K. W.: Contribution of wastewater treatment plant effluents to nutrient dynamics in aquatic systems: a review, Environ. Manage., 44, 205–217, https://doi.org/10.1007/s00267-009-9309-5, 2009.
Christian, R. R., Boyer, J. N., and Stanley, D. W.: Multi-year distribution patterns of nutrients within the Neuse River Estuary, North Carolina, Mar. Ecol. Prog. Ser., 71, 259–274, 1991.
Coastal Emergency Risks Assessment: https://cera.coastalrisk.live/, last access: 9 September 2022.
Davis, S. E., Cable, J. E., Childers, D. L., Coronado-Molina, C., Day, J. W., Hittle, C. D., Madden, C. J., Reyes, E., Rudnick, D., and Sklar, F.: Importance of Storm Events in Controlling Ecosystem Structure and Function in a Florida Gulf Coast Estuary, J. Coast. Res., 204, 1198–1208, https://doi.org/10.2112/03-0072r.1, 2004.
D'Sa, E. J., Joshi, I. D., Liu, B., Ko, D. S., Osburn, C. L., and Bianchi, T. S.: Biogeochemical Response of Apalachicola Bay and the Shelf Waters to Hurricane Michael Using Ocean Color Semi-Analytic/Inversion and Hydrodynamic Models, Front. Mar. Sci., 6, 523, https://doi.org/10.3389/fmars.2019.00523, 2019.
Denman, E. C., May, P. B., and Moore, G. M.: The Potential Role of Urban Forests in Removing Nutrients from Stormwater, J. Environ. Qual., 45, 207–214, https://doi.org/10.2134/jeq2015.01.0047, 2016.
Dewitz, J. and U.S. Geological Survey: National Land Cover Database (NLCD) 2019 Products (ver. 2.0, June 2021): U.S. Geological Survey data release, USGS [data set], https://doi.org/10.5066/P9KZCM54, 2021.
Diaz, R. J. and Roseberg, R.: Marine betnhic hypoxia: A review of its ecological effects and the behavioural responses of benthic macrofauna, Oceanogr. Mar. Biol., 33, 245–303, 1995.
Duda, A. M.: Municipal point source and agricultural nonpoint source contributions to coastal eutrophication, J. Am. Water Resour. As., 18, 397–407, 1982.
Dupas, R., Delmas, M., Dorioz, J.-M., Garnier, J., Moatar, F., and Gascuel-Odoux, C.: Assessing the impact of agricultural pressures on N and P loads and eutrophication risk, Ecol. Indic., 48, 396–407, https://doi.org/10.1016/j.ecolind.2014.08.007, 2015.
Easterling, D. R., Arnold, J., Knutson, T., Kunkel, K., LeGrande, A., Leung, L. R., Vose, R., Waliser, D., and Wehner, M.: Precipitation change in the United States, Climate Science Special Report: Fourth National Climate Assessment, 1, 207–230, https://doi.org/10.7930/J0H993CC, 2017.
Ensign, S. H., Piehler, M. F., and Doyle, M. W.: Riparian zone denitrification affects nitrogen flux through a tidal freshwater river, Biogeochemistry, 91, 133–150, https://doi.org/10.1007/s10533-008-9265-9, 2008.
Eyre, B. D. and Ferguson, A. J. P.: Denitrification efficiency for defining critical loads of carbon in shallow coastal ecosystems, Hydrobiologia, 629, 137–146, https://doi.org/10.1007/s10750-009-9765-1, 2009.
Gold, A. C., Thompson, S. P., and Piehler, M. F.: Coastal stormwater wet pond sediment nitrogen dynamics, Sci. Total Environ., 609, 672–681, https://doi.org/10.1016/j.scitotenv.2017.07.213, 2017.
Gold, A. C., Thompson, S. P., and Piehler, M. F.: The Effects of Urbanization and Retention-Based Stormwater Management on Coastal Plain Stream Nutrient Export, Water Resour. Res., 55, 7027–7046, https://doi.org/10.1029/2019wr024769, 2019.
Gold, A. C., Thompson, S. P., and Piehler, M. F.: Seasonal Variation in Nitrate Removal Mechanisms in Coastal Stormwater Ponds, Water Resour. Res., 57, e2021WR029718, https://doi.org/10.1029/2021wr029718, 2021.
Goodrich, D. M., Boicourt, W. C., Hamilton, P., and Pritchard, D. W.: Wind-induced destratification in Chesapeake Bay, J. Phys. Oceanogr., 17, 2232–2240, 1987.
Grabowski, J. H., Brumbaugh, R. D., Conrad, R. F., Keeler, A. G., Opaluch, J. J., Peterson, C. H., Piehler, M. F., Powers, S. P., and Smyth, A. R.: Economic Valuation of Ecosystem Services Provided by Oyster Reefs, BioScience, 62, 900–909, https://doi.org/10.1525/bio.2012.62.10.10, 2012.
Groffman, P. M. and Crawford, M. K.: Denitrification Potential in Urban Riparian Zones, J. Environ. Qual., 32, 1144–1149, 2003.
Groffman, P. M., Axelrod, E. A., Lemunyon, J. L., and Sullivan, W. M.: Denitrification in Grass and Forest Vegetated Filter Strips, J. Environ. Qual., 20, 671–674, https://doi.org/10.2134/jeq1991.00472425002000030027x, 1991.
Hampel, J. J., McCarthy, M. J., Reed, M. H., and Newell, S. E.: Short Term Effects of Hurricane Irma and Cyanobacterial Blooms on Ammonium Cycling Along a Freshwater–Estuarine Continuum in South Florida, Front. Mar. Sci., 6, 640, https://doi.org/10.3389/fmars.2019.00640, 2019.
Hill, A. R. and Cardaci, M.: Denitrification and Organic Carbon Availability in Riparian Wetland Soils and Subsurface Sediments, Soil Sci. Soc. Am. J., 68, 320–325, https://doi.org/10.2136/sssaj2004.3200a, 2004.
Hirsch, R. M., Moyer, D. L., and Archfield, S. A.: Weighted regressions on time, discharge, and season (WRTDS), with an application to Chesapeake Bay River Inputs, J. Am. Water Resour. As., 46, 857–880, https://doi.org/10.1111/j.1752-1688.2010.00482.x, 2010.
Hohman, S. P., Smyth, A. R., Bean, E. Z., and Reisinger, A. J.: Internal nitrogen dynamics in stormwater pond sediments are influenced by pond age and inorganic nitrogen availability, Biogeochemistry, 156, 255–278, https://doi.org/10.1007/s10533-021-00843-2, 2021.
Howarth, R. W. and Marino, R.: Nitrogen as the limiting nutrient for eutrophication in coastal marine, Limnol. Oceanogr., 51, 364–376, 2006.
Knutson, T. R., McBride, J. L., Chan, J., Emanuel, K., Holland, G., Landsea, C., Held, I., Kossin, J. P., Srivastava, A. K., and Sugi, M.: Tropical cyclones and climate change, Nat. Geosci., 3, 157–163, https://doi.org/10.1038/ngeo779, 2010.
Knutson, T. R., Sirutis, J. J., Vecchi, G. A., Garner, S., Zhao, M., Kim, H.-S., Bender, M., Tuleya, R. E., Held, I. M., and Villarini, G.: Dynamical Downscaling Projections of Twenty-First-Century Atlantic Hurricane Activity: CMIP3 and CMIP5 Model-Based Scenarios, J. Climate, 26, 6591–6617, https://doi.org/10.1175/jcli-d-12-00539.1, 2013.
Kossin, J. P.: A global slowdown of tropical-cyclone translation speed, Nature, 558, 104–107, https://doi.org/10.1038/s41586-018-0158-3, 2018.
Kunkel, K. E., Easterling, D. R., Kristovich, D. A. R., Gleason, B., Stoecker, L., and Smith, R.: Recent increases in U.S. heavy precipitation associated with tropical cyclones, Geophys. Res. Lett., 37, L24706, https://doi.org/10.1029/2010gl045164, 2010.
Lee, S. Y., Dunn, R. J. K., Young, R. A., Connolly, R. M., Dale, P. E. R., Dehayr, R., Lemckert, C. J., McKinnon, S., Powell, B., Teasdale, P. R., and Welsh, D. T.: Impact of urbanization on coastal wetland structure and function, Austral. Ecol., 31, 149–163, https://doi.org/10.1111/j.1442-9993.2006.01581.x, 2006.
Mallin, M. A., Posesy, M. H., McIver, M. R., Parsons, D. C., Ensign, S. H., and Alphin, T. D.: Impacts and recovery from multiple hurricanes in a piedmont-coastal plain river system: human development of floodplains greatly compounds the impacts of hurricanes on water quality and aquatic life, BioScience, 52, 999–1010, 2002.
Mancino, C. F., Torello, W. A., and Wehner, D. J.: Denitrification losses from Kentucky Bluegrass sod, J. Agron., 80, 148–153, 1988.
McPhillips, L. E., Groffman, P. M., Schneider, R. L., and Walter, M. T.: Nutrient Cycling in Grassed Roadside Ditches and Lawns in a Suburban Watershed, J. Environ. Qual., 45, 1901–1909, https://doi.org/10.2134/jeq2016.05.0178, 2016.
Miller, W. D., Harding, L. W., and Adolf, J. E.: Hurricane Isabel generated an unusual fall bloom in Chesapeake Bay, Geophys. Res. Lett., 33, L06612, https://doi.org/10.1029/2005gl025658, 2006.
Min, S. K., Zhang, X., Zwiers, F. W., and Hegerl, G. C.: Human contribution to more-intense precipitation extremes, Nature, 470, 378–381, https://doi.org/10.1038/nature09763, 2011.
Minaudo, C., Dupas, R., Gascuel-Odoux, C., Roubeix, V., Danis, P.-A., and Moatar, F.: Seasonal and event-based concentration-discharge relationships to identify catchment controls on nutrient export regimes, Adv. Water Resour., 131, 103379, https://doi.org/10.1016/j.advwatres.2019.103379, 2019.
Naden, P., Bell, V., Carnell, E., Tomlinson, S., Dragosits, U., Chaplow, J., May, L., and Tipping, E.: Nutrient fluxes from domestic wastewater: A national-scale historical perspective for the UK 1800–2010, Sci. Total Environ., 572, 1471–1484, https://doi.org/10.1016/j.scitotenv.2016.02.037, 2016.
Nixon, S. W.: Coastal marine eutrophication: A definition, social causes, and future concerns, Ophelia, 41, 199–219, https://doi.org/10.1080/00785236.1995.10422044, 1995.
NOAA, National Data Buoy Center: Station CLKN7-Cape Lookout, NOAA, National Data Buoy Center [data set], https://www.ndbc.noaa.gov/station_page.php?station=clkn7, last access: 9 January 2023.
Onorevole, K. M., Thompson, S. P., and Piehler, M. F.: Living shorelines enhance nitrogen removal capacity over time, Ecol. Eng., 120, 238–248, https://doi.org/10.1016/j.ecoleng.2018.05.017, 2018.
Ortiz-Zayas, J. R., Cuevas, E., Mayol-Bracero, O. L., Donoso, L., Trebs, I., Figueroa-Nieves, D., and McDowell, W. H.: Urban influences on the nitrogen cycle in Puerto Rico, Biogeochemistry, 79, 109–133, https://doi.org/10.1007/s10533-006-9005-y, 2006.
Paerl, H. W., Crosswell, J. R., Van Dam, B., Hall, N. S., Rossignol, K. L., Osburn, C. L., Hounshell, A. G., Sloup, R. S., and Harding, L. W.: Two decades of tropical cyclone impacts on North Carolina's estuarine carbon, nutrient and phytoplankton dynamics: implications for biogeochemical cycling and water quality in a stormier world, Biogeochemistry, 141, 307–332, https://doi.org/10.1007/s10533-018-0438-x, 2018.
Paerl, H. W., Hall, N. S., Hounshell, A. G., Luettich Jr., R. A.,, Rossignol, K. L., Osburn, C. L., and Bales, J.: Recent increase in catastrophic tropical cyclone flooding in coastal North Carolina, USA: Long-term observations suggest a regime shift, Sci. Rep., 9, 10620, https://doi.org/10.1038/s41598-019-46928-9, 2019.
Paerl, H. W., Hall, N. S., Hounshell, A. G., Rossignol, K. L., Barnard, M. A., Luettich, R. A., Rudolph, J. C., Osburn, C. L., Bales, J., and Harding, L. W.: Recent increases of rainfall and flooding from tropical cyclones (TCs) in North Carolina (USA): implications for organic matter and nutrient cycling in coastal watersheds, Biogeochemistry, 150, 197–216, https://doi.org/10.1007/s10533-020-00693-4, 2020.
Pérez-Villalona, H., Cornwell, J. C., Ortiz-Zayas, J. R., and Cuevas, E.: Sediment Denitrification and Nutrient Fluxes in the San José Lagoon, a Tropical Lagoon in the Highly Urbanized San Juan Bay Estuary, Puerto Rico, Estuaries Coasts, 38, 2259–2278, https://doi.org/10.1007/s12237-015-9953-3, 2015.
Petrovic, A. M.: The fate of nitrogenous fertilizers applied to turfgrass, J. Environ. Qual., 19, 1–14, 1990.
Piehler, M. F. and Smyth, A. R.: Habitat-specific distinctions in estuarine denitrification affect both ecosystem function and services, Ecosphere, 2, 1–17, https://doi.org/10.1890/es10-00082.1, 2011.
Pinckney, J. L., Paerl, H. W., Harrington, M. B., and Howe, K. E.: Annual cycles of phytoplankton community-structure and bloom dynamics in the Neuse River Estuary, North Carolina, Mar. Biol., 131, 371–381, 1998.
Raciti, S. M., Burgin, A. J., Groffman, P. M., Lewis, D. N., and Fahey, T. J.: Denitrification in suburban lawn soils, J. Environ. Qual., 40, 1932–1940, https://doi.org/10.2134/jeq2011.0107, 2011.
R Core Team: R: A Language and Environment for Statistical Computing, R Foundation for Statistical Computing, Vienna, Austria, https://www.R-project.org/ (last access: 10 November 2023), 2021.
Reisinger, A. J., Groffman, P. M., and Rosi-Marshall, E. J.: Nitrogen cycling process rates across urban ecosystems, FEMS Microbiol. Ecol., 92, fiw198, https://doi.org/10.1093/femsec/fiw198, 2016.
Rosenzweig, B. R., Groffman, P. M., Zarnoch, C. B., Branco, B. F., Hartig, E. K., Fitzpatrick, J., Forgione, H. M., and Parris, A.: Nitrogen regulation by natural systems in “unnatural” landscapes: denitrification in ultra-urban coastal ecosystems, Ecosyst. Health Sust., 4, 205–224, https://doi.org/10.1080/20964129.2018.1527188, 2018.
Rudek, J., Paerl, H. W., Mallin, M. A., and Bates, P. W.: Seasonal and hydrological control of phytoplankton nutrient limitation in the lower Neuse River Estuary, North Carolina, Mar. Ecol. Prog. Ser., 75, 133–142, 1991.
Sebastian, A., Gori, A., Blessing, R. B., van der Wiel, K., and Bass, B.: Disentangling the impacts of human and environmental change on catchment response during Hurricane Harvey, Environ. Res. Lett., 14, 124023, https://doi.org/10.1088/1748-9326/ab5234, 2019.
Seitzinger, S., Harrison, J. A., Böhlke, J. K., Bouwman, A. F., Lowrance, R., Peterson, B., Tobias, C., and Drecht, G. V.: Denitrification across landscapes and waterscapes: A synthesis, Ecol. Appl., 16, 2064–2090, 2006.
Seitzinger, S. P.: Linkages between organic matter mineralization and denitrification in eight riparian wetlands, Biogeochemistry, 25, 19–39, 1994.
Smyth, A. R., Piehler, M. F., Grabowski, J. H., and Frid, C.: Habitat context influences nitrogen removal by restored oyster reefs, J. Appl. Ecol., 52, 716–725, https://doi.org/10.1111/1365-2664.12435, 2015.
Sun, H., Jiang, J., Cui, L., Feng, W., Wang, Y., and Zhang, J.: Soil organic carbon stabilization mechanisms in a subtropical mangrove and salt marsh ecosystems, Sci. Total Environ., 673, 502–510, https://doi.org/10.1016/j.scitotenv.2019.04.122, 2019.
U.S. Geological Survey: Layer: 12-digit HU (Subwatershed) (ID: 6), USGS [data set], https://hydro.nationalmap.gov/arcgis/rest/services/wbd/MapServer/6, (last access: 9 May 2022), 2019.
U.S. Geological Survey: Neuse River Near Fort Barnwell, NC – 02091814, Water Data for the Nation, U.S. Geological Survey [data set] https://waterdata.usgs.gov/monitoring-location/02091814/#parameterCode=00065&period=P7D&showMedian=true, last access: 9 January 2023.
Velinsky, D. J., Paudel, B., Quirk, T., Piehler, M., and Smyth, A.: Salt Marsh Denitrification Provides a Significant Nitrogen Sink in Barnegat Bay, New Jersey, J. Coastal. Res., 78, 70–78, https://doi.org/10.2112/si78-007.1, 2017.
Wang, H., Marshall, C. W., Cheng, M., Xu, H., Li, H., Yang, X., and Zheng, T.: Changes in land use driven by urbanization impact nitrogen cycling and the microbial community composition in soils, Sci. Rep., 7, 44049, https://doi.org/10.1038/srep44049, 2017.
Wang, W., Haver, D., and Pataki, D. E.: Nitrogen budgets of urban lawns under three different management regimes in southern California, Biogeochemistry, 121, 127–148, https://doi.org/10.1007/s10533-013-9942-1, 2013.
Wasson, K., Jeppesen, R., Endris, C., Perry, D. C., Woolfolk, A., Beheshti, K., Rodriguez, M., Eby, R., Watson, E. B., Rahman, F., Haskins, J., and Hughes, B. B.: Eutrophication decreases salt marsh resilience through proliferation of algal mats, Biol. Conserv., 212, 1–11, https://doi.org/10.1016/j.biocon.2017.05.019, 2017.
Wengrove, M. E., Foster, D. L., Kalnejais, L. H., Percuoco, V., and Lippmann, T. C.: Field and laboratory observations of bed stress and associated nutrient release in a tidal estuary, Estuar. Coast. Shelf S., 161, 11–24, https://doi.org/10.1016/j.ecss.2015.04.005, 2015.
Wetz, M. S. and Paerl, H. W.: Estuarine Phytoplankton Responses to Hurricanes and Tropical Storms with Different Characteristics (Trajectory, Rainfall, Winds), Estuaries Coasts, 31, 419–429, https://doi.org/10.1007/s12237-008-9034-y, 2008.
Wobus, C., Gutmann, E., Jones, R., Rissing, M., Mizukami, N., Lorie, M., Mahoney, H., Wood, A. W., Mills, D., and Martinich, J.: Climate change impacts on flood risk and asset damages within mapped 100-year floodplains of the contiguous United States, Nat. Hazards Earth Syst. Sci., 17, 2199–2211, https://doi.org/10.5194/nhess-17-2199-2017, 2017.